Abstract
Self-replicating RNA viruses have been commonly used for preventive and therapeutic interventions in the fields of infectious diseases and cancers. Both RNA viruses with single-stranded RNA genomes of positive and negative polarity have been utilized. Expression of viral surface proteins from self-replicating RNA virus vectors has elicited strong immune responses and provided protection against challenges with lethal doses of pathogens in various animal models using recombinant viral particles, RNA replicons, or plasmid-based replicon vectors. Similarly, immunization with self-replicating RNA virus vectors expressing tumor antigens has induced tumor-specific antibody (Ab) responses, inhibited tumor growth, eradicated tumors, and protected immunized animals against tumor challenges. Clinical trials have demonstrated good safety and tolerance of self-replicating RNA viruses. Although the number of clinical trials is low, robust immune responses and protection against challenges with pathogens and tumor cells have been achieved. The Ervebo vaccine against Ebola virus disease has been approved by both the European Medicines Agency (EMA) and the US Food and Drug Administration (FDA).
Keywords
Self-amplification, recombinant particles, RNA replicons, DNA replicons, vaccines, infectious diseases, cancerIntroduction
Recombinant DNA technology has completely changed drug discovery and vaccine development by making it possible to specifically target signaling pathways and individual genes, and express genes of interest as biomedicines or use as immunogens for vaccines. It has opened the possibilities for structure-based drug design, gene therapy, and precisely targeted vaccine development. Instead of relying on only large-scale compound screening, an area that in itself has seen major progress through automation and miniaturization, structure-based and computer-aided drug and vaccine design has made it possible to evaluate efficacy and safety in silico. However, any drug discovery and vaccine development still heavily rely on in vitro and in vivo research.
Viral and non-viral vectors have played a central role in drug discovery, gene therapy, and vaccine development. Although non-viral vector systems have the advantages of fast and simple large-scale production as well as high biosafety standards, their delivery efficacy is inferior in comparison to viral vectors [1]. Viral vectors, on the other hand, provide superior delivery, but higher production costs and potential safety hazards have raised some concerns [2]. A large repertoire of viral vectors including adenoviruses (Ads), adeno-associated viruses (AAVs), alphaviruses, flaviviruses, herpes simplex viruses (HSVs), lentiviruses (LVs), retroviruses (RVs), measles viruses (MVs), rhabdoviruses, Newcastle disease viruses (NDVs), poxviruses, and picornaviruses, has been engineered for gene therapy and vaccine applications [3]. However, the focus of this review will be on self-replicating RNA viruses.
Among self-replicating RNA viruses, mainly alphaviruses, flaviviruses, MVs and rhabdoviruses have been applied for vaccine development and gene therapy [4]. The various expression systems for self-replicating RNA viruses are summarized and their applications as delivery vehicles for prophylactic use for infectious diseases are described. In the context of cancer, both preventive and therapeutic applications are possible. In addition to cancer vaccines, which directly aim at the expression of tumor-associated antigens (TAAs) for vaccine development, delivery, and expression of toxic, suicide, and anti-tumor genes can generate tumor regression, eradicate tumors, and provide the cure for the disease. Cancer immunotherapy has become an important approach especially since modern technologies do not rely on protein-based delivery, but gene delivery vectors such as self-replicating RNA viruses can produce the immunostimulatory proteins in vivo. These aspects are described in the review.
Self-replicating RNA vectors
The common feature of self-replicating RNA viruses is their single-stranded RNA (ssRNA) genome. However, there is a significant difference between alphaviruses and flaviviruses possessing a genome of positive polarity and MVs and rhabdoviruses with a negative-strand RNA genome. The RNA from self-replicating positive ssRNA viruses is directly translated in the cytoplasm of infected host cells, while viruses with a genome of negative polarity require an intermediate positive-strand copy translation before can occur (Figure 1). The most important feature of all self-replicating RNA viruses is their capability of high-level RNA amplification directly in the cytoplasm of host cells. For example, it has been estimated that alphaviruses can accumulate approximately 106 RNA copies per cell [5]. In addition to the amplification of a large number of RNA copies, the expression levels of the gene of interest (GoI) are very high as the expression is driven by strong viral promoters. However, the expression is transient lasting approximately 5–7 days due to RNA degradation [6]. The transient nature of expression is ideal for vaccine development and cancer therapy, but for the treatment of chronic diseases requiring long-term expression, other vector systems should be considered. The application of self-replicating RNA virus vectors is not restricted to recombinant viral particles as also naked or liposome-encapsulated RNA replicons and layered DNA/RNA plasmid vectors can be used. The different self-replicating RNA virus systems are described below.
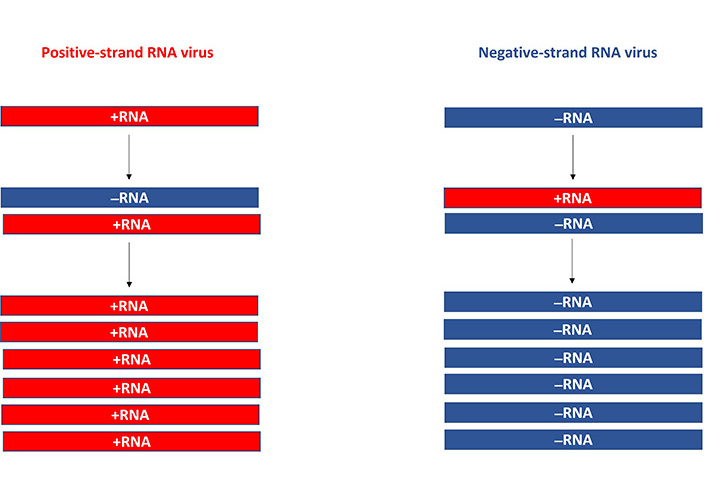
Positive- and negative-strand RNA viruses. The viral RNA of positive-strand RNA viruses is directly translated into the cytoplasm (right) and new positive-strand RNA copies are transcribed from a negative-strand RNA template (left). For negative-strand RNA, messenger RNA (mRNA) transcription needs to take place before translation
Alphavirus vectors
Alphavirus-based expression systems have been developed for Semliki Forest virus (SFV) [7], Sindbis virus (SIN) [8], and Venezuelan equine encephalitis virus (VEE) [9]. The basic alphavirus expression system comprises an expression vector and helper vector as illustrated for SFV in Figure 2. The expression vector carries the four SFV non-structural protein 1–4 (nsP1–4) genes, the subgenomic 26S promoter generally driving the expression of the SFV structural genes but has been replaced by the GoI. In vitro transcribed RNA from linearized SFV DNA plasmids can be directly used for transfection of host cells and recombinant protein expression. For the generation of replication-deficient viral particles, a helper vector carrying the SFV structural genes expressing the capsid (C), a precursor of E2 and E3 envelope (Env) proteins (p62), the small 6K protein, and the E1 Env protein is used for in vitro transcription and electroporation/transfection of baby hamster kidney (BHK-21) cells [7]. The produced recombinant SFV particles can efficiently infect mammalian and non-mammalian cells, primary cells in culture (primary neurons, rat hippocampal slices), and animals in in vivo studies. However, due to the presence of the packaging signal only in the RNA of the expression vector, no new SFV particles will be generated. If replication-proficient viral particles are preferred, which is often the case for oncolytic viruses (described below), SFV vectors with a second subgenomic 26S promoter followed by the GoI can be introduced into the full-length RNA genome either downstream of the non-structural or structural protein genes. The replication-proficient SFV vector can also be used for RNA delivery. Alternatively, the replacement of the SP6 RNA polymerase promoter by a cytomegalovirus (CMV) promoter enables the use of the SFV expression vector directly as a plasmid DNA-based transfection system of mammalian cells [10]. Delivery of both RNA- and DNA-based alphavirus vectors can be improved by encapsulation in nanoparticles and especially RNA degradation can be reduced by the encapsulation procedure [11]. Lipid nanoparticle (LNP) formulations have generally been based on ionizable lipids, phospholipids, cholesterol, and polyethylene glycol coated (PEGylated) lipids providing a particle size of 120–160 nm in diameter [12]. In more detail, LNPs contain the ionizable cationic lipid 1,2-dilinoleyloxy-3-dimethylaminopropane (DLinDMA), the cationic lipid 1,2-dioleoyl-3-trimethylammonium-propane (DOTAP), 1,2-diastearoyl-sn-glycero-3-phosphocholine (DSPC) and 1,2-dimyristoyl-sn-glycero-3-phosphoethanolamine-N-[methoxy(polyethylene glycol)-2000] (ammonium salt, PEG-DMG 2000) [13].
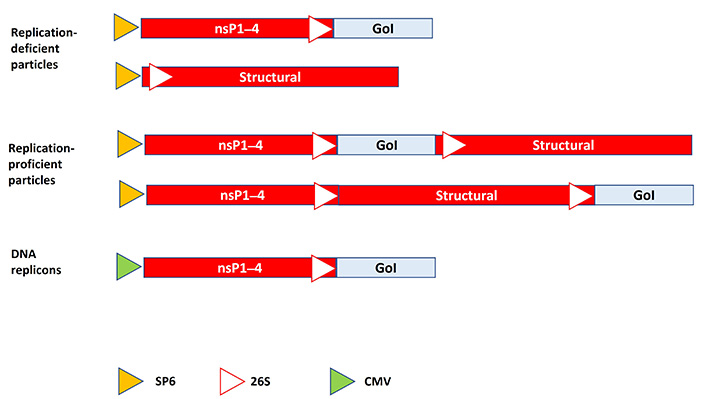
Schematic presentation of alphavirus expression systems. Replication-deficient alphavirus particles are generated from expression vector and helper vector DNA, which are in vitro transcribed and co-transfected/electroporated into mammalian cells for production of recombinant particles. Replication-proficient alphavirus particles are generated from the full-length alphavirus genome containing a second 26S subgenomic promoter and the GoI. In vitro transcribed RNA is transfected/electroporated into host cells for virus production. DNA replicon vector can be transfected into host cells for expression of the GoI. No virus progeny is produced. SP6: SP6 RNA polymerase promoter; 26S: alphavirus 26S subgenomic promoter
Flavivirus vectors
Flaviviruses such as Kunjin virus (KUN) [14], West Nile virus (WNV) [15], yellow fever virus (YFV) [16], dengue virus (DENV) [17], and tick-borne encephalitis virus (TBEV) [18] have been used for the engineering of expression systems. Moreover, expression vectors for bovine viral diarrhea virus (BVDV) [19] and classic swine fever virus (CSFV) have been constructed [20]. Typically, KUN expression vectors have been engineered to carry the GoI between the first 60 nucleotides of the C20 KUN core protein and the last 22 codons of the KUN E22 Env protein [14] (Figure 3). Because the GoI is part of a larger polyprotein, the introduction of the 18 amino acids long foot-and-mouth disease virus protease sequence allows the removal of the KUN flanking sequences from the GoI product [21]. To facilitate the production of KUN particles, packaging cell lines have been engineered [22].
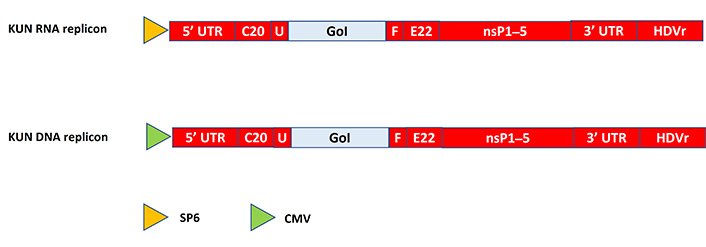
Schematic presentation of flavivirus expression systems. The GoI is introduced between C20 and E22 in frame with the downstream viral polyprotein. In the RNA-based system, the SP6 RNA polymerase promoter is used. For use of the DNA-based system, the SP6 promoter is replaced by a CMV promoter. 5’ UTR: 5’ untranslated region; C20: first 20 amino acids of KUN C protein; E22: last 22 amino acids of KUN E protein; U: mouse ubiquitin sequence; F: foot-and-mouth disease virus 2A autoprotease; HDVr: hepatitis delta virus ribozyme
MVs vectors
In contrast to the positive-strand self-replicating RNA viruses, the negative-strand RNA viruses including paramyxoviruses such as MVs have required the engineering of packaging systems and helper cell lines [23]. In the context of MVs expression vector systems, the GoI is introduced either between the phosphoprotein (P) and the matrix (M) protein or between the hemagglutinin (HA) and the large (L) protein (Figure 4). For MVs, different rescue methods have been developed, such as engineering of stable human embryonic kidney 293 (HEK293) cell lines expressing the MV-nucleoprotein (N), MV-P and T7 polymerase for co-transfection with a plasmid expressing the full-length MV genome, the GoI and the T7 polymerase driven MV-L expression [24, 25]. In another strategy, the MV-N, MV-P and MV genomes are expressed from DNA plasmids in HEK293 cells superinfected with a replication-deficient vaccinia virus [VV; modified VV Ankara (MVA)] providing the T7 polymerase [26]. An MV rescue technology has also been established for CMV-driven DNA plasmids expressing the MV genome and the RNA-dependent RNA polymerase (RdRp) complex [27].
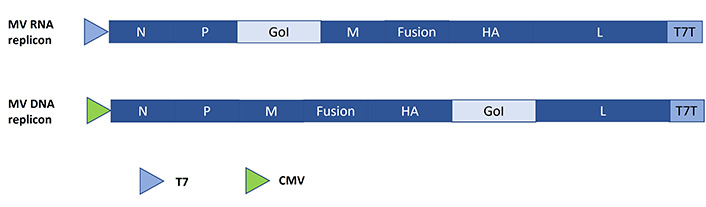
Schematic presentation of MV expression systems. The GoI is introduced either between the P and the M protein or between the HA and the L protein. T7: T7 RNA polymerase promoter; T7T: T7 terminator
Rhabdovirus vectors
In the context of negative-strand rhabdoviruses, expression vectors have been constructed for vesicular stomatitis virus (VSV) [28] and rabies virus (RABV) [29]. Reverse genetics based on a recombinant VV has been applied for VSV [30] and RABV [31] engineering. Additionally, a VV-free system has been developed, where the VSV N, P and L genes are driven by a T7 polymerase promoter and an internal ribosome entry site (IRES) [32].
Typically, both pseudotyped and recombinant VSV particles have been engineered [28, 33]. Generally, pseudotyped VSV particles are generated by replacing the VSV glycoprotein (G) with another Env protein and VSV pseudovirus particles can selectively infect host cells but generate no virus progeny (Figure 5A) [28]. However, fully infectious VSV particles can be generated in mammalian producer cell lines [33]. An alternative strategy comprises the insertion of the GoI between the M and G genes and/or the G and L genes in the VSV genome (Figure 5B). VSV particles have also demonstrated oncolytic activity, preferentially replicating in and killing tumor cells without causing damage to normal cells. Similarly, the Mariba virus has been engineered as an oncolytic vector for cancer therapy [34]. In the context of RABV vectors, the GoI is inserted between the N and P genes or between the G and L genes [35, 36] (Figure 5C).
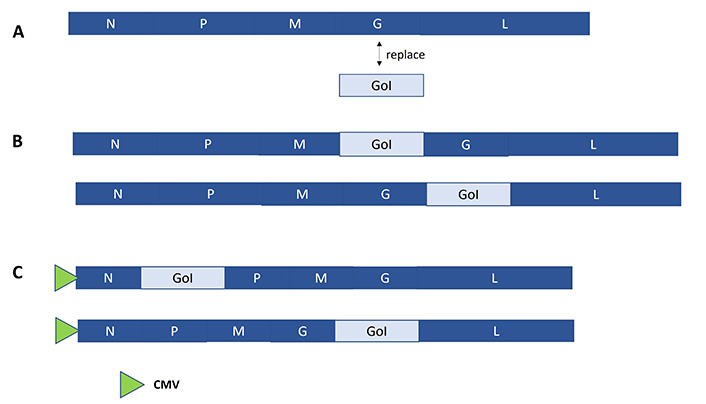
Schematic presentation of rhabdovirus expression systems. A. VSV pseudovirus system, where the VSV G protein is replaced by the GoI; B. recombinant VSV expression system, where the GoI can be inserted between the M and G proteins, or between the G and L proteins; C. RABV system, where the GoI is introduced between the N and P proteins, or the G and L proteins
Self-replicating RNA for infectious diseases
Self-replicating RNA viruses have been frequently used for prophylactic approaches to infectious diseases including viral, bacterial, and parasitic pathogens [4]. However, as very few studies on bacterial and parasitic vaccines have been conducted with self-replicating RNA, they are only briefly summarized here. Swiss Webster mice immunized with SFV particles expressing the Bacillus anthracis protective antigen (PA) elicited PA-specific immunoglobulin G (IgG) and neutralizing Ab responses and gave some protection against B. anthracis challenges [37]. Moreover, BALB/c mice immunized with SFV particles expressing the Brucella abortus translation initiation factor 3 (IF3) elicited IF3-specific immune responses and protected mice from B. abortus challenges [38]. Alphavirus-based malaria vaccines have been developed by expressing the Plasmodium falciparum Pf332 antigen from SFV particles [39]. Immunization of BALB/c mice elicited T-helper 1 (Th1)-biased immune responses. Moreover, BALB/c mice were immunized with SIN particles expressing the Plasmodium yoelii circumsporozoite (CS) protein class I major histocompatibility complex-restricted-9-mer epitope SYVpSAEQI, resulting in epitope-specific CD8+ T-cell responses and protection against malaria [40].
As vaccines against pathogenic viruses have previously been presented and discussed in numerous reviews listing indications and applications of self-replicating RNA viruses [4, 41], the focus in this review is on delivery methods, achieved immune responses, and potential protection as described below and summarized in Table 1. Moreover, clinical studies are summarized separately and listed in Table 2.
Self-replicating RNA viruses and examples of preclinical studies on infectious diseases
Delivery method | Indication | Outcome | Reference |
---|---|---|---|
Recombinant particles | |||
VSV-CHIKV-Env/ZIKV-ME | CHIKV | Protection against CHIKV in mice | [42] |
VSV-CHIKV-Env/ZIKV-ME | ZIKV | Protection against CHIKV in mice | [42] |
VEE-E2/E3 FCSdel | VEE | Full protection in mice & macaques | [43] |
WEE-E2/E3 FCSdel | WEE | Protection in mice, partial protection in macaques | [43] |
EEE-E2/E3 FCSdel | EEE | Full protection in mice | [43] |
MV-CHIKV C/Env | CHIKV | Viremia protection in macaques | [44] |
VSV-LASV-GPC | LHF | Protection in guinea pigs & macaques | [45] |
MV-LASV-GPC | LHF | Protection in macaques | [46] |
YFV-LASV-GPC | LHF | Protection in guinea pigs | [47] |
YFV-LASV-GPC | LHF | Failure to protect marmosets | [48] |
VEE-LASV-GPC/LASV-N | LHF | Protection in guinea pigs | [49] |
VEE-LASV-GPC | LHF | Protection in inbred CBA/J mice | [50] |
VEE-EBOV-G | EVD | Protection in guinea pigs | [49] |
KUN-EBOV-G D637L | EVD | Protection in 75% of primates | [51] |
VSV-EBOV-G | EVD | Protection against EBOV-Makuna | [52] |
VSV-EBOV-G | EVD | Protection against ZEBOV | [53] |
VEE-DENV-E85 | DF | Protection against DENV in mice | [54] |
MV-DENV-ED3 | DF | Partial protection in mice | [55] |
MV-ZIKV-E (MV-E2) | ZIKV | Protection against lethal ZIKV in mice | [56] |
MV-E2 + MV-NIS | ZIKV | ZIKV clearance from reproductive tract | [56] |
MV-HIV-1 Gag gp160 | HIV/AIDS | Strong cellular & humoral response | [57] |
SFV-HIV Env | HIV/AIDS | Superior response to conDNA, recEnv | [58] |
SFV-HIV-Env-Gag-Pol-RT | HIV/AIDS | Superior compared to RNA replicons | [59] |
MV AIK-C IFVA-HA | Influenza | Protection in cotton rats | [60] |
VSVΔG IFVA-HA/NA | Influenza | Protection in mice | [61] |
VSV IFVA-HAfl | Influenza | 100% protection after single dose | [62] |
VEE IFVA-HA | Influenza | Protection against IFVA in chickens | [63] |
SIN-E2S1-M2e | Influenza | 100% protection in mice | [64] |
CSFV-IFVA-HA + N | Influenza | Superior immunogenicity to replicon RNA | [65] |
VEE-SARS-CoV S | SARS | Protection against SARS in mice | [67] |
VSVΔG MERS-CoV S | MERS | Neutralizing Ab and T-cell responses | [68] |
MV-SARS-CoV-2 S | COVID-19 | Th1-biased Ab and T-cell responses | [69] |
VSV-SARS-CoV-2 S | COVID-19 | Neutralizing Abs, protection in mice | [70] |
VSVΔG-SARS-CoV-2 S | COVID-19 | Protection in Syrian golden hamsters | [71] |
SIN-SARS-CoV-2 S + αOX40 | COVID-19 | Protection against SARS-CoV-2 in mice | [72] |
BVDV-PEDV S | PEDV | PEDV specific Abs, neutralization of PEDV | [73] |
Attenuated virus | |||
YFV (CYD-TDV) | DF | Good safety, no toxicity concerns | [74] |
CHIKV-NoLS RNA-LNP | CHIKV | Local protection against CHIKV in mice | [75] |
VEE-RABV-G | RABV | Protection against RABV in mice | [76] |
RNA replicons | |||
VEE-ZIKV-ME | ZIKV | Protection in mice with 10 ng RNA | [78] |
SFV-HIV-Env-Gag-Pol-Rev | HIV/AIDS | Inferior compared to SFV particles | [59] |
SFV-HIV Env | HIV/AIDS | Env-specific Abs in 80% of mice | [79] |
VEE-HIV-1 gp140 | HIV/AIDS | Improved RNA stability in mice | [80] |
VEE-HIV-1 gp140 | HIV/AIDS | Superior to VEE particles, rec Env | [81] |
CSFV-IFVA-HA/N | Influenza | Inferior to CSFV particles | [65] |
CSFV-IFVA-HA/N-PEI | Influenza | PEI formulation superior to naked RNA | [65] |
SFV-IFVA HA | Influenza | Protection against IFVA in mice | [82] |
SFV-IFVA-HA | RSV | Protection against RSV in mice | [82] |
SFV-IFVA-HA | TBEV | Protection against TBEV in mice | [82] |
VEE-IFVA-HA | Influenza | 64x less RNA replicon than mRNA needed | [84] |
VEE-SARS-CoV-2-LNPs | COVID-19 | Dose-dependent Abs, SARS-CoV-2 neutralization | [12] |
VEE (LUNAR-COV19) | COVID-19 | Protection in ACE2 transgenic mice | [85] |
Plasmid DNA replicons | |||
VEE V4020 | VEE | Protection against wildtype VEE in mice | [86] |
VEE V4020 | VEE | Protection against wildtype VEE in macaques | [87] |
SFV-HIV Env/Gag-Pol-Nef | HIV/AIDS | Superior with MVA boost | [88] |
ACE2: angiotensin-converting enzyme 2; AIDS: acquired immunodeficiency syndrome; αOX40: OX40 immunostimulatory Ab; CHIKV: chikungunya virus; CHIKV-Env: CHIKV E3-E2-6K-E1 Env polyprotein; COVID-19: coronavirus disease 2019; CYD-TDV: chimeric YFV-based tetravalent DENV vaccine; DF: dengue fever; EBOV: Ebola virus; ED3: DENV Env protein domain III; EEE: eastern equine encephalitis virus; EVD: EBOV disease; FCSdel: furin cleavage site deletion; GPC: glycoprotein complex; HAfl: full-length HA; HIV: human immunodeficiency virus; IFVA: influenza virus A; LASV: Lassa fever virus; LHF: Lassa hemorrhagic fever; M2e: M2 protein ectodomain; ME: membrane Env; MERS: Middle East respiratory syndrome; NA: neuraminidase; NIS: sodium iodide symporter; NoLS: nucleolar localization signals; PEDV: porcine epidemic diarrhea virus; PEI: polyethylenimine; RSV: respiratory syncytial virus; SARS: severe acute respiratory syndrome; SARS-CoV-2: SARS-coronavirus-2; WEE: western equine encephalitis virus; ZEBOV: Zaire EBOV; ZIKV: Zika virus
Self-replicating RNA viruses and examples of clinical trials on infectious diseases
Vaccine candidate | Indication | Status | Reference |
---|---|---|---|
Recombinant particles | |||
MV-LASV (V182-001) | LF | Phase I: 52 volunteers, in progress | NCT04055454 |
VSV-ZEBOV | EVD | Phase III: good vaccine efficacy | [89] |
VSV-ZEBOV | EVD | Phase III: good vaccine efficacy | [90] |
VSV-ZEBOV | EVD | Approval by the FDA & EMA | [91] |
VEE-HIV-Gag (AVX101) | HIV/AIDS | Phase I: two studies terminated | [94] |
MV-SARS-CoV-2 S (TMV-083) | COVID-19 | Phase I: weak response, terminated | NCT04497298 |
VSV-SARS-CoV-2 S (V590) | COVID-19 | Phase I: weak response, terminated | NCT04469786 |
VSVΔG-SARS-CoV-2 S (IIBR-100) | COVID-19 | Phase I/II: study in progress | NCT04608305 |
VSVΔG-SARS-CoV-2 S (IIBR-100) | COVID-19 | Phase IIb/III: study registered | NCT04990466 |
Attenuated virus | |||
CYD-TDV | DHF | Vaccine efficacy in several trials | [92] |
CYD-TDV (Dengvaxia®) | DHF | Regulatory approval | [93] |
RNA replicons | |||
SARS-CoV-2 S RNA-LNP (COVAC1) | COVID-19 | Phase I/II: no results available | ISRCTN17072692 |
DHF: dengue hemorrhagic fever; EMA: European Medicines Agency; FDA: Food and Drug Administration; LF: Lassa fever
Recombinant particles for infectious diseases
Clearly, the most common approach has been the utilization of recombinant viral particles in different forms. In this context, replication-deficient vectors have been frequently used. For example, a chimeric VSV vector was engineered by replacing the VSV G protein with the CHIKV-Env and the ZIKV ME glycoproteins [42]. A single administration of 1 × 107 chimeric VSV particles induced neutralizing Ab responses to both CHIKV and ZIKV and protected wild-type and interferon (IFN) receptor-deficient A129 mice against both CHIKV and ZIKV challenges. In another approach, the removal of the furin cleavage site (FCS) between the E2 and E3 Env proteins in VEE, WEE and EEE generated infectious but replication-deficient viral particles [43]. Co-immunization of mice with VEE, WEE, and EEE or with individual particles elicited robust immune responses and protected mice from subcutaneous or aerosol challenges with any of the three alphaviruses. Likewise, cynomolgus macaques showed complete protection against challenges with VEE and EEV, but only partial protection against WEE was established. Related to MVs, expression of CHIKV C and Env proteins from an MV vector elicited strong immune responses and protected macaques from viremia [44].
In the context of LHF, immunization of guinea pigs and macaques with VSV particles expressing the LASV GPC resulted in protection against challenges with LASV strains from Liberia, Mali, and Nigeria [45]. Likewise, a single immunization of macaques with 1 × 106 plaque forming units (pfu) of recombinant MV-LASV-GPC particles provided protection [46]. Additionally, YFV vectors expressing the LASV-GPC showed protection in 80% of immunized guinea pigs [47], but immunization of marmosets failed to prevent LASV infections [48]. Among alphaviruses, VEE particles expressing LASV-GPC or N protected guinea pigs from challenges with the LASV Josiah strain [49]. Similarly, the VEE-LASV-GPC protected inbred CBA/J mice from challenges with the LASV LP and Josiah strains [50]. In the case of EBOV, VEE particles expressing EBOV-G protected guinea pigs from EBOV challenges [49]. Furthermore, KUN-based expression of the EBOV-G D637L mutant, showing superior cleavability and shedding compared to wildtype G, provided protection in 75% of immunized nonhuman primates [51]. VSV-based EBOV vaccine candidates have been promising as shown by the immunization of macaques with 5 × 107 VSV-EBOV-G particles, resulting in protection against challenges with EBOV-Makona [52] and ZEBOV [53] strains. VEE particles expressing the ectodomain (ECD) of the DENV Env prevented DENV infection in immunized mice [54]. Partial protection was achieved in mice immunized with MV particles expressing the ED3 [55]. In the context of ZIKV, it was mentioned above that immunization of mice with chimeric VSV particles expressing the precursor membrane-Env of ZIKV (ZIKV-prME) elicited strong immune responses and provided protection against challenges with ZIKV [42]. Moreover, mice immunized with MV particles expressing the ZIKV Env gene were protected against challenges with the nonlethal ZIKV Asian strain PRVABC59 and the lethal African strain MR766 [56]. However, viral clearance from the brain and reproductive tract did not take place. Co-administration of MV-E2 and MV-NIS resulted in complete viral clearance of ZIKV from the female reproductive tract and fetal protection [56].
Vaccine development against HIV/AIDS has been of significant interest and self-replicating RNA viruses are no exception. MV vectors expressing HIV-1 Gag-like particles induced robust cellular and humoral immune responses in mice [57]. Alphaviruses have been frequently used for HIV vaccine development. For instance, immunization of mice with SFV-HIV Env particles resulted in higher Ab titers compared to immunization with conventional plasmid DNA or recombinant Env protein [58]. Furthermore, SFV particles expressing the Indian HIV-1C Env-Gag-Pol-RT genes induced robust T-cell responses, which were superior in comparison to SFV RNA replicon immunization [59].
The seasonal IFV outbreaks have increased the interest in utilizing self-replicating RNA viruses for vaccine development. For example, the recombinant MV AIK-C vaccine candidate carries the IFVA HA gene from the influenza A/Sapporo/107/2013 (H1N1pdm) strain [60]. Immunization of cotton rats with the MV AIK-C IFVA-HA induced robust immune responses and protected rats against challenges with IFVA. Furthermore, the VSV G protein was replaced by the IFVA HA protein from the highly pathogenic avian IFV (HPAIV) A/Vietnam/1203/04 (VN1203) strain and the NA protein of the mouse-adapted H1N1 IFVA/Puerto Rico/8/34 (PR8) in the VSVΔG vector for immunization studies in mice [61]. A single immunization with VSVΔG-H5N1 protected mice from challenges with pathogenic H5N1 strains. Moreover, VSV particles expressing the HAfl were subjected to either a single dose or prime-boost regimens in mice [62]. A single dose of VSV-HAfl was fast-acting, showing 100% protection when administered three days prior to IFVA challenges. It also induced cross-protective H5-specific Ab responses and protection was achieved against challenges with various H5 clade 2 strains. In another approach, a single dose of 1 × 107 pfu of VEE-HA particles provided protection in chickens challenged with the IFVA isolate A/HK/156/97 [63]. Moreover, SIN particles expressing the external domain of the IFVA M2e in the SIN E2 Env protein were subjected to intranasal immunization of mice [64]. All mice vaccinated with SIN-E2S1-M2e particles survived challenges with a virulent IFVA strain, while control animals did not. In the case of CSFV, the IFVA N and HA genes were introduced into the CSFV RepRNA vector [65]. Pigs immunized with a mixture of CSFV-Rep-HA and CSFV-Rep-N particles were compared to CSFV RNA replicon-PEI complexes and naked RNA replicons. Strong HA- and N-specific humoral and cellular responses were detected after immunization with CSFV particles and RNA-PEI formulations. In contrast, naked CSFV RNA replicons elicited only modest immunogenicity. Overall, the strongest immunogenicity was achieved with CSFV particles.
Coronaviruses have gained special attention for vaccine development in light of the current COVID-19 pandemic. Although several viral vector-based vaccines have received emergency use authorization (EUA) and have been extensively reviewed elsewhere [66], the focus of this review will be solely on vaccine candidates based on self-replicating RNA viruses. Prior to the SARS-CoV-2, mice immunized with VEE particles expressing the SARS-CoV spike (S) protein gave protection against challenges with SARS-CoV [67]. In the case of the MERS, the VSVΔG-MERS-CoV S vector was engineered, where the VSV G gene was replaced by the MERS-COV S gene [68]. Intramuscular or intranasal immunizations of rhesus macaques induced neutralizing Ab and T-cell responses. In the context of COVID-19, MV-based expression of SARS-CoV-2 S induced strong Th1-biased Ab and T-cell responses in immunized mice [69]. Moreover, immunization of mice with VSV-SARS-CoV-2 S particles induced neutralizing Ab responses and provided protection against SARS-CoV-2-related pathogenesis [70]. Like MERS-CoV-2 S, the SARS-CoV-2 S gene was introduced into the VSVΔG vector for immunization of Syrian golden hamsters, which elicited potent neutralizing antibodies (Abs) and protected the animals against challenges with SARS-CoV-2 [71]. Recently, co-immunization of SIN particles expressing the SARS-CoV-2 S protein and the αOX40 in C57BL/6J mice elicited long-lasting neutralizing Abs and robust T-cell responses after prime-boost vaccination [72]. Furthermore, the treatment protected mice from SARS-CoV-2.
In addition to human coronaviruses, vaccine development has taken place for animal coronaviruses. Chimeric BVDV particles expressing the PEDV S protein were intramuscularly administered to BALB/c mice, resulting in BVDV- and PEDV-specific Ab responses and neutralization of both BVDV and PEDV [73].
Attenuated viruses for infectious diseases
In another approach, a live-attenuated CYD-TDV has been evaluated in cynomolgus macaques showing good safety with no toxicological concerns after repeated administration [74]. In another approach, the live-attenuated CHIKV-NoLS vaccine candidate was formulated for in vivo RNA delivery in cationic adjuvant formulation 01 (CAF01) liposomes [75]. Immunization of immunocompetent C57BL/6 mice with liposome-delivered CHIKV-NoLS RNA elicited reduced IgM and IgG levels with low CHIKV-neutralizing capacity compared to the original live-attenuated CHIKV-NoLS. The immunization provided local protection in the ipsilateral foot of mice, but not systemic protection against wild-type CHIKV after one injection. In another study, an attenuated hybrid VEE-RABV replicon vector was engineered by replacing all VEE structural proteins with RABV G [76]. Mice immunized with the hybrid vector elicited strong humoral immune responses and were protected against challenges with virulent RABV. Moreover, the VEE-RABV-G was highly attenuated and caused much weaker inflammatory and apoptotic effects in the brain, resulting in significantly lower weight loss and morbidity compared to standard live-attenuated RABV.
RNA replicons for infectious diseases
RNA-based vaccine approaches have been applied long before the current COVID-19 vaccines were developed [77]. The RNA amplification of self-replicating RNA viruses in the host cell cytoplasm has made RNA replicons attractive and potentially superior to synthetic mRNA as vaccine vehicles. Like synthetic mRNA, encapsulation of RNA replicons in LNPs or nanocarrier lipids (NLCs) has improved delivery and reduced RNA degradation. For example, immunization of mice with NLC formulations of VEE-based ZIKV-ME RNA replicons showed complete protection against ZIKV with only a single administration of 10 ng of RNA [78]. In another approach, naked SFV-HIV Env RNA replicons were intramuscularly injected into mice, which induced Env-specific immune responses in 80% of immunized animals [79]. The delivery and stability of VEE-HIV-1 gp140 RNA replicons were improved in mice by encapsulation in cationic nanoemulsion (CNE), which consisted of squalene, DOTAP and sorbitan trioleate [80]. Compared to VEE particles or MF59-adjuvanted HIV gp140 recombinant protein, intramuscular administration of 50 μg of VEE-HIV-1 gp140 RNA-CNE formulations induced stronger immune responses in rhesus macaques [81]. In another study, immunization with as little as 10 μg of naked SFV-HA RNA replicon resulted in protection in 90% of BALB/c mice [82]. Moreover, immunization with SFV-HA RNA replicons provided protection against RSV and TBEV in mice [82]. In another study, the VEE-HA RNA replicon [83] was compared to immunization of mice with synthetic mRNA [84], which confirmed that only 1.25 μg of RNA replicon was needed to establish protection against IFVA challenges compared to 80 μg of synthetic mRNA.
LNP-encapsulated VEE RNA replicons carrying the prefusion-stabilized SARS-CoV-2 S gene have been intramuscularly administered to BALB/c mice, showing strong dose-dependent SARS-CoV-2-specific Ab responses and neutralization of SARS-CoV-2 [12]. Moreover, BALB/c mice immunized with the VEE-based self-transcribing and replicating RNA (STARR)-based vaccine (LUNAR-COV19) expressing the full-length SARS-CoV-2 S protein generated robust neutralizing immune responses [85]. Protection against SARS-CoV-2 was achieved in humanized ACE2 transgenic mice.
Plasmid DNA replicons for infectious diseases
The application of self-replicating RNA viruses based on plasmid DNA has allowed direct use of DNA avoiding safety concerns related to viral vectors and degradation issues with RNA delivery. For example, immunization of BALB/c mice with plasmid DNA replicons of the attenuated VEE V4020 strain-induced strong neutralizing Ab responses and provided protection against wild-type VEE [86]. Furthermore, immunization with VEE V4020 DNA protected cynomolgus macaques from aerosol challenges with wild-type VEE [87]. In another study, BALB/c mice were immunized with SFV DNA replicons expressing the HIV Env and Gag-Pol-Nef fusion protein, which elicited robust immune responses [88]. A single low dose of 0.2 μg of SFV DNA replicons was sufficient for greatly enhanced immune responses when the prime immunization was followed by a heterologous boost with MVA particles expressing HIV Env and Gag-Pol-Nef fusion protein [88].
Clinical trials for infectious diseases
Self-replicating RNA viruses have also been subjected to clinical trials for infectious diseases (Table 2). For example, a randomized, placebo-controlled, dose-finding phase I trial to find the optimal dose of MV-LASV (V182-001) in 52 healthy volunteers is in progress (NCT04055454). Related to EVD, the VSV-EBOV-G vaccine candidate VSV-ZEBOV has been evaluated in 7,651 individuals with suspected EVD in a phase III trial showing good efficacy and protection [89]. Another phase III trial in 4,160 individuals confirmed the efficacy of the VSV-ZEBOV vaccine [90]. Both the US FDA and the EMA have approved VSV-ZEBOV [91]. The YFV-based tetravalent CYD-TDV has been subjected to several clinical trials, showing an overall pooled protective vaccine efficacy of 65.5% [92]. However, the vaccine was substantially more effective against severe DF. The CYD-TDV vaccine (Dengvaxia®) has received regulatory approval in several countries [93]. The VEE-based HIV-1 Gag vaccine AVX101 has been subjected to two phase I double-blind, randomized, placebo-controlled studies in healthy volunteers in the US and South Africa [94]. Due to vaccine instability issues, one trial was stopped. Unfortunately, the second trial had also to be prematurely discontinued due to manufacturing issues and lower immune responses seen in humans compared to findings from preclinical studies.
Among negative-strand self-replicating RNA viruses, the MV-SARS-CoV-2 S vaccine candidate TMV-083 showed disappointingly weak immune responses in a COVID-19 phase I trial in vaccinated volunteers, which led to the termination of the study (NCT04497298). Similarly, despite showing good safety and tolerability profiles, the VSV-SARS-CoV-2 S vaccine candidate V590 showed a weaker immune response than obtained from convalescent COVID-19 patients in a phase I trial, justifying the early discontinuation of the study (NCT04569786). In another approach, a phase I/II clinical study for the VSVΔG-SARS-CoV-2 S vaccine candidate is in progress (NCT04608305). Healthy volunteers will receive a single dose of 5 × 105, 5 × 106, or 5 × 107 pfu of VSVΔG-SARS-CoV-2 (IIBR-100) for the evaluation of the safety, immunogenicity, and potential efficacy of the vaccine candidate. Moreover, a phase IIb/III for IIBR-100 has been registered for a study against an approved COVID-19 vaccine (NCT04990466).
In the context of RNA replicons, a phase I randomized, placebo-controlled, dose-finding phase I/II study for the SARS-CoV-2 S RNA-LNP vaccine candidate COVAC1 has been registered (ISRCTN17072692). So far, no results have been published.
Self-replicating RNA for cancer therapy
In contrast to the prophylactic applications for infectious diseases, cancers can be subjected to both preventive and therapeutic approaches. Several self-replicating RNA viruses have demonstrated both protection against tumor challenges and eradication of pre-existing cancers. A special emphasis will be dedicated to oncolytic viruses, which have demonstrated great promise in tumor therapy [95]. Typically, oncolytic viruses efficiently replicate in tumor cells and kill them without causing much harm to normal cells, making them attractive therapeutic delivery vehicles. Naturally, oncolytic variants have been identified for NDV [96]. Moreover, oncolytic viruses have been engineered for HSV, Ads, VVs and reoviruses. In the case of self-replicating RNA viruses, attenuated MV strains have been discovered [97], oncolytic VSV vectors have been engineered [98], and the naturally oncolytic M1 alphavirus has been identified [99]. Due to a large number of preclinical studies, selected examples are presented below and summarized in Table 3. Moreover, results from clinical trials are discussed and listed in Table 4.
Self-replicating RNA viruses and examples of preclinical studies on cancer
Delivery method | Cancer | Outcome | Reference |
---|---|---|---|
Recombinant particles | |||
SFV-endostatin | GBM | Tumor regression, superior to RV delivery | [100] |
SFV-IL-18 + rec IL-12 | GBM | Th1-biased response, anti-tumor immunity | [101] |
VSVΔ-CHIKV Env | GBM | Selective infection, prolonged survival in mice | [102] |
SFV4-miRT124 | Glioma | Tumor growth inhibition, prolonged survival | [104] |
SFV-1L-12 + LVR01 | Breast | Inhibition of metastases, long-term survival | [105] |
MV-HPV-16 L1 | Cervical | Humoral immune response, neutralizing Abs | [106] |
MV-HPV-16 L1 | Cervical | Robust immune responses in primates | [107] |
VSV-CRPV-E1/E2/E6/E7 | Cervical | 96.6% of papilloma volume, tumor eradication | [108] |
VSV-HPV-16 E7 | Cervical | T-cell responses, tumor volume reduction | [109] |
VEE-HPV-16 E7 | Cervical | T-cell responses, protection against tumors | [110] |
SFVenh-HPV E6E7 | Cervical | Complete tumor eradication in mice | [111] |
KUN-GM-CSF | Colon | Tumor regression, cure of 50% of mice | [112] |
SFV-VEGFR-2 | Colon | Inhibition of tumor growth and metastases | [113] |
SFV-VEGFR-2 + SFV-IL-4 | Colon | Enhanced effect of co-administration | [113] |
MV-GM-CSF | Colon | Complete remission in one third of mice | [114] |
SFV-EGFP | Lung | Complete tumor regression of 3 out 7 mice | [115] |
SIN-LacZ | Lung | Complete tumor remission, long-term survival | [116] |
VSV-IFNβ | Lung | Tumor regression, cure of 30% of mice | [117] |
KUN-GM-CSF | Melanoma | Tumor regression, cure of > 50% of mice | [112] |
VEE-TRP-2 | Melanoma | Anti-tumor activity, prolonged survival | [118] |
VEE-TRP-2 + CTLA-4 | Melanoma | Tumor regression in 50% of mice | [119] |
VEE-TRP-2 + GITR | Melanoma | Complete tumor regression in 90% of mice | [119] |
SIN-Luc | Hematologic | Tumor targeting, prolonged survival in mice | [120] |
SIN + α4-1BB mAb | Lymphoma | Complete tumor eradication in mice | [121] |
SFV-A20 mAb | Lymphoma | Antitumor protection, prolonged survival in mice | [122] |
MV-αFR | Ovarian | Reduced tumor volume, improved survival | [123] |
MV-CEA/MV-NIS | Ovarian | Superior tumor growth inhibition | [124] |
SIN-IL-12 + irinotecan | Ovarian | Combination superior for prolonged survival | [125] |
SFV-OVA + VV-OVA | Ovarian | Enhanced anti-tumor activity | [126] |
MV-SLAMBlind | Pancreatic | Significant suppression of tumor growth | [127] |
MV-CEA | Prostate | Delay in tumor growth, prolonged survival | [128] |
VEE-PSMA | Prostate | PSMA-specific immune response | [129] |
VEE-STEAP | Prostate | T-cell responses, prolonged survival | [130] |
VEE-PSCA | Prostate | Long-term survival in 90% of mice | [131] |
Oncolytic viruses | |||
MV-GFP/CEA/NIS | Glioma | Cytotoxicity in GSCs, prolonged survival in mice | [132] |
SFV VA-EGFP | Glioma | Long-term survival in 16 out 17 mice | [133] |
M1 + doxorubicin | Breast | 100-fold oncolytic activity with doxorubicin | [99] |
MV-SLAMBlind | Breast | Strong oncolytic activity in mice | [134] |
SFV VA-EGFP | Lung | Superior to Ad-based delivery | [135] |
MV Hu-191 | Lung | Tumor growth inhibition, prolonged survival | [136] |
MV Schwarz | Lung | Prevention of uncontrolled tumor growth | [137] |
MV-CEA Edmonston | Lung | Tumor regression in mice | [138] |
YFV-OVA | Melanoma | Protection against tumor challenges in mice | [139] |
MV-L-16 | Melanoma | Tumor cell killing, prevention of tumor growth | [140] |
VSV-LCMV-G | Melanoma | Tumor and metastases regression | [141] |
VSV-LCMV-G + Rux | Ovarian | Tumor cell killing, enhanced tumor regression | [142] |
VSVΔM51 | Pancreatic | Superior tumor regression to Sendai virus & RSV | [143] |
VSVΔM51-GFP + Gem | Pancreatic | Superior tumor regression with Gem | [144] |
MV + MuV | Prostate | Superior oncolytic activity with combination | [145] |
VSVΔM51-GFP | Prostate | Eradication of tumors, prolonged survival | [146] |
VSV-LCMV-G | Prostate | Long-term remission of tumors and metastases | [147] |
RNA replicons | |||
SFV-LacZ | Colon | Tumor protection, regression. > survival | [148] |
KUN-Mpt | Melanoma | CD8+ T-cell responses, protection against B16 | [149] |
Plasmid DNA replicons | |||
SIN-gp100-IL-18 | GBM | Protective and therapeutic effects, survival | [150] |
SIN-HER2/neu | Breast | Tumor inhibition, superior with Ad-HER2/neu | [151] |
SIN-HER2/neu | Breast | 80% less DNA used compared to plasmid DNA | [152] |
SFV-HPV E6-E7 | Cervical | Eradication of 85% of tumors | [153] |
SFV-VEGFR-2/IL-12 + SFV-survivin/β-hCG Ag | Melanoma | Superior tumor growth inhibition, prolonged survival after co-administration | [154] |
CEA: carcinoembryonic antigen; CRPV: cottontail rabbit papillomavirus; CTLA-4: anti- cytotoxic T lymphocyte antigen-4; EGFP: enhanced green fluorescent protein; GBM: glioblastoma; Gem: gemcitabine; GFP: green fluorescent protein; GITR: anti-glucocorticoid-induced TNF family-related gene; GM-CSF: granulocyte-macrophage colony-stimulating factor; GSCs: glioma stem cells; HER2: human epidermal growth factor receptor 2; HPV: human papillomavirus; IL: interleukin; L-16: Leningrad-16; LCMV: lymphocytic choriomeningitis virus; Luc: luciferase: mAb: monoclonal Ab; Mpt: murine polyepitope; MuV: mumps virus; OVA: ovalbumin; PSCA: prostate stem cell antigen; PSMA: prostate-specific membrane antigen; Rux: ruxolitinib; SLAM: signaling lymphocyte activation molecule; STEAP: six-transmembrane epithelial antigen of the prostate; TRP-2: tyrosine-related protein-2; VEGFR-2: vascular epithelial growth factor receptor-2; αFR: alpha-folate receptor; β-hCG: beta-human chorionic gonadotropin
Self-replicating RNA viruses and examples of clinical trials on cancers
Vaccine candidate | Cancer | Status | Reference |
---|---|---|---|
VEE-HER2 ECD-TM (VRP-HER2) | Breast | Phase I: 1 PR, 2 SD, good tolerance | [155] |
VEE-HER2 ECD-TM (VRP-HER2) | Breast | Phase II: study in progress | [155] |
SFVEnh-HPV E6/E7 (Vvax001) | Cervical | Phase I: immunogenicity of all 12 patients | [156] |
VEE-CEA | Colon | Phase I: prolonged survival | [157] |
VEE-CEA | Colon | Phase II: study in progress | [158] |
MV-CEA | Ovarian | Phase I: dose-dependent, SD in 14/21 patients | [159] |
VEE-CEA | Pancreatic | Phase I: prolonged overall survival | [159] |
VEE-PSMA | Prostate | Phase I: safe, but weak immunogenicity | [160] |
PR: partial response; SD: stable disease; SFVEnh: SFV vector with translation enhancement signal from C protein; TM: transmembrane; VRP: virus replicon particle
Recombinant particles for cancers
In the context of brain tumors, SFV particles expressing endostatin showed superior tumor regression and a decrease in intratumoral vascularization in a B16 brain tumor mouse model compared to RV-based endostatin expression [100]. Moreover, dendritic cells (DCs) transduced with SFV-IL-18 particles combined with recombinant IL-12 induced Th1-biased immune responses and elicited anti-tumor immunity in a B16 brain tumor mouse model [101]. Selective infection and elimination of brain tumor cells were achieved with the chimeric VSVΔG-CHIKV vector, where the VSV G protein was replaced by the CHIKV E3-E2-6K-E1 polyprotein [102].
Moreover, VSVΔG-CHIKV demonstrated targeting of intracranial xenografts derived from melanoma patients, while normal cells showed no or low infection rates. Administration of VSVΔG-CHIKV prolonged the survival of tumor-bearing mice for over 100 days. Targeting brain cancers, especially with replication-proficient viral particles, have raised some concern related to the neurotropic nature of many viruses such as alphaviruses [103]. For this reason, neuron-specific micro-RNA miRT124 sequences were inserted into the genome of the replication-competent SFV4 virus [104]. This manipulation altered the viral tropism resulting in SFV4-miRT124 targeting CT-2A orthotopic gliomas, significant tumor growth inhibition and prolonged survival in intraperitoneally injected C57BL/6 mice. In the case of breast cancer, complete inhibition of lung metastases and long-term survival in 90% of animals were observed in 4T1 tumor-bearing mice immunized with SFV-IL-12 particles and the aroC Salmonella typhimurium strain LVR01 [105]. The combination therapy was superior compared to the administration of either SFV-IL-12 or LVR01 alone.
Self-replicating RNA viruses have also been applied for immunizations against HPVs. For example, immunization of MV-susceptible IFN alpha receptor (IFNAR)/CD46 transgenic mice with recombinant MV particles expressing the HPV-16 L1 C protein elicited strong humoral immune responses and neutralizing Abs against L1 [106]. Moreover, MV-HPV 16 L1 C particles have been compared to immunizations with recombinant HPV-16 L1 and HPV-18 L1 protein vaccines produced in Pichia pastoris yeast cells [107]. Immunization of non-human primates elicited strong immune responses for both virus- and protein-based vaccines. The CRPV E1, E2, E6 and E7 proteins have been expressed from VSV vectors for evaluation in rabbits [108]. Immunization of rabbits with VSV-CRPV-E1, E2, E6, and E7 significantly reduced papilloma volumes, with VSV-CRPV-E7 showing a superior reduction in papilloma volume of 96.9% and complete eradication of existing tumors. In another study, TC-1 tumor-bearing C57BL/6 mice were intramuscularly immunized with VSV-HPV E7, which induced HPV-16 E7-specific T-cell responses and reduced tumor volumes 10-fold [109]. Additionally, VEE particles expressing the HPV-16 E7 protein elicited CD8+ T-cell responses and protected immunized C57BL/6 mice from tumor challenges [110]. Additionally, the HPV E6-E7 fusion protein was expressed from the SFVenh vector, providing enhanced transgene expression due to the presence of the translation enhancer signal from the SFV C gene [111]. Immunization of C57BL/6 mice with SFVenh-HPV E6-E7 particles generated complete eradication of established tumors.
Self-replicating RNA viruses have also been validated for colon cancer treatment. KUN particles expressing the GM-CSF were intratumorally administered to BALB/c mice with CT26 colon tumors, which elicited CD8+ T-cell responses, resulting in tumor regression and cure of 50% of immunized mice [112]. Moreover, immunization of BALB/c mice with SFV particles expressing the VEGFR-2 resulted in inhibition of tumor growth, reduction in tumor angiogenesis and prevention of the spread of metastases [113]. When SFV-IL-4 particles were co-administered with SFV-VEGFR-2 particles, the VEGFR-2-specific Ab responses were enhanced, and the survival of mice was prolonged [113]. In another study on colon cancer, mice with MC38cea colon adenocarcinomas were immunized with MV-GM-CSF particles, which delayed tumor progression and increased the survival time of mice [114]. Intratumoral administration resulted in complete remission in one-third of mice and tumor re-engraftment was rejected. In the context of lung cancer, SFV-EGFP particles efficiently killed human H358a non-small cell lung cancer (NSCLC) cells and inhibited the growth of H358a spheroids through SFV-based apoptotic action and induction of caspase activity [115]. Immunization of nu/nu mice with SFV-EGFP particles showed complete tumor regression in three out of seven nu/nu mice implanted with H358a lung tumors. In another study, complete tumor remission and long-term survival were observed in CT26. CL25 tumor-bearing mice immunized with SIN-LacZ particles [116]. Moreover, intratumoral administration of VSV particles expressing VSV-IFNβ resulted in tumor regression, prolonged survival, and cure in 30% of immunized mice with syngeneic LM2 lung tumors [117].
Several studies have involved melanoma, such as immunization of B16-OVA tumor-bearing C57BL/6 mice with KUN-GM-CSF particles, which showed substantial tumor regression and cure of more than 50% of immunized animals [112]. Furthermore, immunization of mice with B16 melanoma tumors with VEE particles expressing the TRP-2 elicited humoral responses, demonstrated anti-tumor activity, and prolonged survival of immunized mice [118]. The combination of VEE-TRP-2 particles with the antagonist CTLA-4 mAb resulted in tumor regression in 50% of immunized mice, while complete tumor regression was seen in 90% of mice after co-administration of VEE-TRP-2 particles and the agonist anti-glucocorticoid-induced GITR mAb [119].
In the case of hematological cancers, SIN vectors expressing Luc have demonstrated good infection efficacy of malignant BW5147 mouse hematopoietic T-cells resulting in SIN-induced apoptosis [120]. Intraperitoneal administration of SIN particles targeted tumors and substantially prolonged survival in tumor-bearing CH3HXAKR mice. In another approach, combination therapy of SIN particles and the anti-4-1BB mAb showed complete tumor eradication in a BALB/c A20 B cell lymphoma model [121]. Moreover, a non-cytopathic SFV vector has been utilized for the overexpression of the idiotypic mouse follicular lymphoma-derived A20 mAb in BHK cells [122]. The purified A20 mAb was conjugated to keyhole limpet hemocyanin for immunization of BALB/c mice, which induced antitumor responses and prolonged survival of immunized animals.
In the context of ovarian cancer, a tumor-targeting MV vector containing the single-chain Ab variable fragment (scFv) specific for the αFR has been engineered [123]. Intratumoral MV-αFR injection of mice implanted with ovarian SKOV3ip.1 tumors showed a reduction in tumor volume and improved overall survival. MV-CEA and MV-NIS particles have also been applied for immunization of mice carrying SKOV3ip.1 tumors [124]. Although immunization with MV-CEA or MV-NIS generated inhibition of tumor growth in mice, the effect was significantly better after co-administration of MV-CEA and MV-NIS. In another approach, severe combined immunodeficiency (SCID) mice simultaneously immunized with SIN-IL-12 particles and treated with the CPT-11 topoisomerase inhibitor irinotecan showed substantial inhibition of growth of aggressive ES2 human ovarian tumors and long-term survival of mice [125]. A prime-boost regimen with SFV-OVA particles followed by immunization with VV-based OVA expression elicited OVA-specific CD8+ T-cell responses and accelerated anti-tumor activity in C57BL/6 mice implanted with murine ovarian surface epithelial carcinoma (MOSEC) [126].
Pancreatic cancer has been targeted by engineering an MV vector selectively unable to use the SLAM [127]. Immunization of SCID mice carrying KLM1 and Capan-2 pancreatic tumor xenografts with MV-SLAMBlind particles demonstrated significant suppression of tumor growth.
In the context of prostate cancer, intratumoral administration of MV-CEA particles in a prostate PC-3 mouse model resulted in a significant delay in tumor growth and prolonged survival of mice [128]. Furthermore, VEE particles expressing the PSMA induced robust PSMA-specific immune responses in BALB/c and C57BL/6 mice [129]. In another study, VEE-based expression of the STEAP elicited CD8+ T-cell responses against a newly defined STEAP epitope and prolonged overall survival in prophylactic and therapeutic mouse models [130]. Likewise, VEE-based expression of the PSCA in transgenic adenocarcinoma of the mouse prostate (TRAMP) mice resulted in long-term survival in 90% of mice [131].
Oncolytic viruses for cancer
As many of the oncolytic viruses described for cancer therapy are attenuated strains, it makes sense to describe them in the same paragraph. Oncolytic MV vectors engineered to express GFP, CEA, and NIS demonstrated efficient replication and induction of cytotoxicity in GSCs [132]. Furthermore, MV-NIS administration to nude mice showed significantly prolonged survival. In another study, oncolytic replication-proficient SFV VA-EGFP particles were intravenously administered to BALB/c mice expressing firefly Luc in a subcutaneous orthotopic human glioma model [133]. A single administration of SFV VA-EGFP particles resulted in complete inhibition of intracranial firefly Luc expression and long-term survival in 16 out of 17 mice.
The oncolytic M1 alphavirus has been evaluated for triple-negative breast cancer (TNBC), the most aggressive subtype of breast cancer [99]. The oncolytic activity of M1 in a TNBC mouse model was further enhanced by 100-fold by co-administration of doxorubicin. In another approach, MV-SLAMBlind particles showed strong oncolytic activity against human breast cancer xenografts in immunodeficient mice [134]. Moreover, subcutaneous administration of cynomolgus macaques with MV-SLAMBlind particles resulted in no measles-related clinical symptoms, indicating its safe use for breast cancer therapy [135]. In the case of lung cancer, superior survival was achieved after local immunization of nude mice carrying A549 lung adenocarcinoma with oncolytic SFV VA-EGFP particles in comparison to conditionally replicating Ad Ad5-Delta24TK-GFP particles [135]. However, systemic administration was unable to elicit significant immune responses with either vector system. Moreover, the oncolytic MV Hu-191 strain showed efficient suppression of tumor growth and prolongation of survival of immunized Lewis lung carcinoma (LLC)-bearing CH57BL/6 mice [136]. Additionally, uncontrolled growth of lung and colorectal adenocarcinomas was prevented in nude mice immunized with the live-attenuated oncolytic MV Schwarz strain [137]. In another study, the potent killing of lung cancer cell lines and tumor regression in nude mice was achieved after immunization with the oncolytic MV Edmonston strain expressing CEA [138]. In the case of melanoma, a live-attenuated YFV strain expressing the chicken OVA cytotoxic T lymphocyte (CTL) epitope SIINFEKL induced CD8+ lymphocyte responses and protected immunized C57BL/6 mice from challenges with B16-OVA or B16F0 cells [139]. In another approach, the oncolytic MV L-16 strain showed the potential killing of tumor cells and prevented tumor growth in the mel Z mouse melanoma model [140]. Moreover, the pseudotyped VSV expressing the LCMV G generated tumor regression in subcutaneous A375 xenograft and B16-OVA syngeneic mouse tumor models and further regression of lung metastases after systemic administration [141]. The MV-LCMV-G vector also showed oncolytic activity in ovarian cancer cell lines and in vivo in the A2780 ovarian mouse tumor model [142]. Combination therapy with the JAK1/2 inhibitor Rux further increased tumor regression. Oncolytic VSVΔM51 particles have also been compared to Sendai virus and RSV in the highly aggressive pancreatic ductal adenocarcinoma (PDAC) [143]. Although the susceptibility of VSV varied among PDAC cells, its oncolytic activity was superior compared to the Sendai virus or RSV. Furthermore, the oncolytic VSV-ΔM51-GFP vector generated reduced tumor growth in mice implanted with PDAC xenografts, which was improved by co-administration of Gem [144].
Prostate cancer has been targeted by oncolytic self-replicating RNA viruses. For example, anti-tumor activity and prolonged survival in the PC-3 prostate cancer mouse model were seen by co-administration of oncolytic MV and MuV [145]. Co-administration of MV and MuV was superior compared to immunization with either MV or MuV alone. In another approach, the oncolytic VSV-ΔM51-GFP vector efficiently was replicated in DU145 and PC-3 prostate tumor cells and induced their death [146]. Immunization of nude mice with VSV-ΔM51-GFP resulted in selective eradication of malignant cells and prolonged the survival of animals. Moreover, the oncolytic VSV-LMCV-G vector efficiently infected and killed several prostate cancer cell lines [147]. In vivo, intratumoral and intravenous administration of VSV-LCMV-G generated long-term remission of subcutaneous tumors and bone metastases in DU145 and 22Rv1 prostate tumor mouse models.
RNA replicons for cancer
The classic example of RNA replicon immunization dates back to 1999 [148]. As little as 0.1 μg SFV-LacZ RNA elicited antigen-specific CD8+ T-cell responses and protected immunized mice against challenges with CT26 colon tumors. Therapeutic efficacy was also seen, where tumor regression and prolonged survival were obtained in mice with pre-existing tumors. In another study, BALB/c mice were intramuscularly immunized with naked KUN RNA replicons expressing the Mpt [149]. Immunization with 30 μg of KUN-Mpt RNA elicited CD8+ T-cell responses and provided protection against B16 melanoma cell challenges.
DNA replicons for cancer
The therapeutic effect of SIN DNA replicon-based expression of human gp100, a melanoma antigen, and mouse IL-18 has been evaluated in the B16-gp100 mouse model [150]. Immunization of mice resulted in both enhanced protective and therapeutic effects on brain tumors and significantly prolonged the survival of mice. In the context of breast cancer, Ad particles and SIN DNA replicons expressing the HER2/neu gene were subjected to immunization of BALB/c mice [151]. Both Ad-HER2/neu and SIN-HER2/neu DNA replicons inhibited the growth of A2L2 tumor growth in BALB/c mice, but only when the immunization took place before the tumor challenge. Moreover, the robust Ab responses and tumor protection seen for intradermal administration of SIN-HER2/neu DNA replicons in BALB/c mice could be achieved with 80% less replicon DNA than conventional plasmid DNA [152].
A prime-boost strategy of SIN-HER2/neu DNA replicon immunization followed by Ad-HER2/neu administration significantly extended the survival of immunized mice. In the context of HPV vaccines, mice were immunized with SFV DNA replicons expressing the HPV E6-E7 genes [153]. Combining intradermal SFV-HPV E6-E7 DNA replicon administration with electroporation resulted in the eradication of 85% of tumors. Moreover, it was demonstrated that only an SFV DNA replicon dose of 0.05 μg, equivalent to a 200-fold lower dose than conventional plasmid DNA, was sufficient for achieving therapeutic efficacy. In another study, mice implanted with B16 melanoma tumors were immunized with one SFV DNA replicon expressing VEGFR-2 and IL-12 and another expressing survivin and the β-hCG antigens [154]. Immunization of mice with either SFV DNA replicon inhibited tumor growth, but co-administration of both SFV DNA replicons showed superior tumor growth inhibition and prolonged survival of mice.
Clinical trials for cancers
In the context of clinical trials, several studies have been conducted with self-replicating RNA viruses (Table 4). For example, expression of the extracellular and TM domains of HER2 (HER ECD and TM) from VEE particles (VRP-HER2) was subjected to a phase I clinical trial in stage IV HER2-overexpressing breast cancer patients [155]. The outcome was good tolerance, PR in one patient and SD in two other patients. Furthermore, a phase II study on VRP-HER2 combined with pembrolizumab was initiated in 39 HER2-positive breast cancer patients [156]. Related to cervical cancer, SFVEnh-HPV E6/E7 particles (Vvax001) were evaluated in 12 patients with a history of cervical intraepithelial neoplasia receiving three immunizations of 5 × 105, 5 × 106, 5 × 107, or 2.5 × 108 infectious particles in a phase I trial [156]. The immunization was safe and well-tolerated, eliciting HPV-specific immune responses in all 12 patients. VEE particles expressing CEA were administered four times every three weeks to patients with stage IV colorectal cancer [157]. The vaccination-induced antigen-specific effector T-cells and prolonged survival in patients. The study has been further expanded to include stage III colorectal cancer patients. In another trial, patients with taxol- and platinum-refractory recurrent ovarian cancer (RROC) were immunized with MV-CEA particles [158]. Intraperitoneal administration of MV-CEA was well-tolerated and resulted in dose-dependent biological activity in patients who had previously received heavy treatment. SD was observed in 14 out of 21 patients. In another approach, VEE-CEA particles were applied for repeated intramuscular administration to pancreatic cancer patients in a phase I trial [159]. The immunization elicited clinically relevant T-cell responses, which resulted in cellular toxicity against tumor cells and prolongation of the overall survival of patients. A phase I clinical trial has also been conducted in patients with metastatic castration resistant prostate cancer (mCRPC) [160]. Patients were subjected to immunization with 0.9 × 107 or 3.6 ×107 international units (IU) of VEE-PSMA particles. The vaccination was safe and well-tolerated. However, the PSMA-specific immune responses were weak, which will require further optimization of dosing and vector delivery.
Conclusions
Self-replicating RNA viruses have found a variety of applications to target both infectious diseases and cancers. Application of alphavirus, flavivirus, MV and rhabdovirus expression systems have elicited robust immune responses against antigens for both infectious agents and tumors. In many cases, protection against challenges with lethal pathogens and tumor cells has been obtained in rodents and primates. In the case of cancers, tumor regression and eradication, even complete cure, have been achieved in various animal models. Also, clinical trials have resulted in protection against for instance EBOV, and PR and SD have been observed in cancer patients. Vaccines against EVD (Ervebo) and DF (Dengvaxia®) have been approved for human use.
However, issues related to vector use and efficacy still remain. Based on the numerous studies conducted, it is not possible to indicate which viral system is the best. Efficacy for vaccines against both infectious diseases and cancers has been described for all self-replicating RNA viruses tested. It is even difficult to rank recombinant particles, RNA replicons, and DNA-based vectors, as it seems that even comparison of the expression systems for different indications varies. One issue of concern has been the discrepancy between efficacy in rodent models and humans in clinical trials. It is obviously well documented for any vaccine development that responses seen in animal models are not always reproducible in clinical settings in humans. Particularly related to cancer, the induced tumor models in rodents do not reflect the cases of spontaneously occurring cancers in humans. In this context, it might be advantageous to use canines with naturally occurring cancers, which would be better reflect the situation in cancer patients.
In comparison to other vaccine development methods, it is justified to say that self-replicating RNA viruses are attractive vectors. The immune responses and protection are generally very good. The RNA amplification generates high levels of RNA, which can result in better efficacy and the need for lower doses of RNA or DNA as has been demonstrated. Self-replicating RNA viruses have also been used for combination therapy with other viral vectors, immunostimulatory Abs (αOX40), and other drugs (Rux, Gem and irinotecan). The flexibility of using different forms of viral particles such as replication-deficient and -proficient, live-attenuated and oncolytic viruses, as well as naked or encapsulated RNA replicons and DNA replicons, is a great asset for vaccine development. How does the future look for therapeutic and prophylactic applications of self-replicating RNA viruses? As with all drug and vaccine development, delivery, efficacy and safety are the three key elements for success. Delivery has always been a strong asset for viral vectors and self-replicating RNA viruses present no exception. However, the broad host range of alphaviruses, flaviviruses, MVs and rhabdoviruses, especially their neurotropism have been of some concern. This has resulted in intensive research efforts to engineer targeted viral vectors, which has included not only identifying naturally occurring oncolytic viruses, but also the design of vectors targeting tumors and antigen presenting cells. Specific targeting is one aspect of enhanced safety, which has also been addressed by using replication-deficient particles and RNA replicons. The short-term high-level transgene expression is ideal for both vaccine development and cancer therapy. However, the application of ssRNA molecules sensitive to degradation has raised some concerns about delivery and efficacy, which has been addressed by the formulation of nanoparticles. Furthermore, the success in both cancer therapy and most recently in RNA-based vaccine development against the COVID-19 pandemic has further been encouraging for self-replicating RNA viruses, becoming major players in the future development of medicines and vaccines.
Abbreviations
Ab: | antibody |
Ads: | adenoviruses |
AIDS: | acquired immunodeficiency syndrome |
BVDV: | bovine viral diarrhea virus |
C: | capsid |
CEA: | carcinoembryonic antigen |
CHIKV: | chikungunya virus |
CMV: | cytomegalovirus |
COVID-19: | coronavirus disease 2019 |
CRPV: | cottontail rabbit papillomavirus |
CSFV: | classic swine fever virus |
CYD-TDV: | chimeric yellow fever virus-based tetravalent dengue virus vaccine |
DENV: | dengue virus |
DF: | dengue fever |
E2: | Zika virus envelope |
EBOV: | Ebola virus |
ECD: | ectodomain |
EEE: | eastern equine encephalitis virus |
EGFP: | enhanced green fluorescent protein |
Env: | envelope |
EVD: | Ebola virus disease |
FCSdel: | furin cleavage site deletion |
G: | glycoprotein |
GBM: | glioblastoma |
Gem: | gemcitabine |
GFP: | green fluorescent protein |
GM-CSF: | granulocyte-macrophage colony-stimulating factor |
GoI: | gene of interest |
GPC: | glycoprotein complex |
HA: | hemagglutinin |
HAfl: | full-length hemagglutinin |
HER2: | human epidermal growth factor receptor 2 |
HIV: | human immunodeficiency virus |
HPV: | human papillomavirus |
IFN: | interferon |
IFVA: | influenza virus A |
IgG: | immunoglobulin G |
IL: | interleukin |
KUN: | Kunjin virus |
L: | large |
LASV: | Lassa fever virus |
LCMV: | lymphocytic choriomeningitis virus |
LHF: | Lassa hemorrhagic fever |
LNP: | lipid nanoparticle |
Luc: | luciferase |
M: | matrix |
M2e: | matrix protein 2 protein ectodomain |
mAb: | monoclonal antibody |
ME: | membrane envelope |
MERS: | Middle East respiratory syndrome |
Mpt: | murine polyepitope |
mRNA: | messenger RNA |
MuV: | mumps virus |
MVA: | modified vaccinia virus Ankara |
MVs: | measles viruses |
N: | nucleoprotein |
NIS: | sodium iodide symporter |
NoLS: | nucleolar localization signals |
nsP1–4: | non-structural protein 1–4 |
OVA: | ovalbumin |
P: | phosphoprotein |
PDAC: | pancreatic ductal adenocarcinoma |
PEDV: | porcine epidemic diarrhea virus |
PEI: | polyethylenimine |
pfu: | plaque forming units |
PR: | partial response |
PSMA: | prostate-specific membrane antigen |
RABV: | rabies virus |
RSV: | respiratory syncytial virus |
Rux: | ruxolitinib |
RVs: | retroviruses |
S: | spike |
SARS: | severe acute respiratory syndrome |
SARS-CoV-2: | severe acute respiratory syndrome coronavirus-2 |
SD: | stable disease |
SFV: | Semliki Forest virus |
SFVEnh: | Semliki Forest virus vector with translation enhancement signal from C protein |
SIN: | Sindbis virus |
SLAM: | signaling lymphocyte activation molecule |
ssRNA: | single-stranded RNA |
STEAP: | six-transmembrane epithelial antigen of the prostate |
TBEV: | tick-borne encephalitis virus |
Th1: | T-helper 1 |
TM: | transmembrane |
TRP-2: | tyrosine-related protein-2 |
VEE: | Venezuelan equine encephalitis virus |
VEGFR-2: | vascular epithelial growth factor receptor-2 |
VRP: | virus replicon particle |
VSV: | vesicular stomatitis virus |
VV: | vaccinia virus |
WEE: | western equine encephalitis virus |
YFV: | yellow fever virus |
ZEBOV: | Zaire Ebola virus |
ZIKV: | Zika virus |
αFR: | alpha-folate receptor |
αOX40: | OX40 immunostimulatory antibody |
Declarations
Author contributions
The author contributed solely to the work.
Conflicts of interest
The author declares that he has no conflicts of interest.
Ethical approval
Not applicable.
Consent to participate
Not applicable.
Consent to publication
Not applicable.
Availability of data and materials
Not applicable.
Funding
Not applicable.
Copyright
© The Author(s) 2022.