Abstract
Vaccination has made an enormous contribution to global health. Treatment resistance for infectious diseases is growing quickly, and chemotherapeutic toxicity in cancer means that vaccines must be made right away to save humanity. But subunit vaccinations alone don’t give enough strong and long-lasting protection against infections that can kill. Nanoparticle (NP)-based delivery vehicles, such as dendrimers, liposomes, micelles, virosomes, nanogels, and microemulsions, offer interesting ways to get around the problems with traditional vaccine adjuvants. The nanovaccines (50–250 nm in size) are most efficient in terms of tissue targeting, staying in the bloodstream for a long time. Nanovaccines can improve antigen presentation, targeted delivery, stimulation of the body’s innate immune system, and a strong T-cell response without putting people at risk. This can help fight infectious diseases and cancers. Also, nanovaccines can be very helpful for making cancer treatments that use immunotherapy. So, this review highlights the various types of NPs used in the techniques that have worked in the new paradigm in viral vaccinology for infectious diseases. It gives a full rundown of the current NP-based vaccines, their potential as adjuvants, and the ways they can be delivered to cells. In the future, the best nanovaccines will try to be more logically designed, have more antigens in them, be fully functionalized, and be given to the right people.
Keywords
Adjuvant, cancer, infection, nanoparticle, nanovaccine, non-neutralizing antibody, neutralizing antibodyIntroduction
Infectious diseases still worry people all over the world the most. Conventional ways of getting immune responses from vaccines don’t work well enough to control new and re-emerging pathogens. Nanoparticle (NP) systems have shown promise as ways to effectively deliver antigens as vaccines or boost immune responses as adjuvants. NP-based vaccines may be able to get around these problems because they are flexible and can be changed to keep antigens from breaking down too quickly, make it easier for them to get into cells, and cause long-lasting immunity to infectious diseases.
NPs can come from nature or be made by humans. NPs can be made in two ways: physically or chemically. NPs are small, uniform pieces of common materials that are smaller than 100 nm [1–3]. NP research is a part of nanotechnology, which has moved into modern biological and medical fields and could soon change everything from the size of electronics to diseases diagnosis tools. NPs are also used in biomedical applications of nanotechnology. Once upon a time, the main ways to treat cancer were chemotherapy, radiation therapy, and surgery.
As the COVID-19 pandemic shows, viral infections that cause pandemics and chronic diseases are the main cause of devastating health and economic effects around the world [4]. Immuno-prophylaxis through mass immunization with vaccines has been shown to be an effective way to control these viral infections. This is because the development of different types of vaccines has been successful and has recently sped up, thanks to the use of advanced biotechnological techniques in both the upstream and downstream processing of these products. The choice of delivery systems, formulations, dosage form, and route of administration is important not only for the effectiveness of immunization, but also for the stability of vaccines, the number of doses a patient needs, how easy it is for the patient to take the vaccine, and the logistics of mass immunization. But there is still a lot of work to be done to improve the safety and effectiveness of vaccines in terms of their stability, how often they are given, how easy they are for patients to use, and how they are set up for mass immunization.
This review has addressed recent developments in NP vaccine delivery systems, the important physiological processes involved in delivering vaccines, and highlighted the challenges that need to be overcome to make NP-based vaccines and adjuvants available to the public in the new paradigm in viral vaccinology for infectious diseases. During the development of these systems, the manufacturing and regulatory requirements were also considered to make sure they would work in clinical trials and on the market. This article looks at nanovaccines in depth, from how they are made to how they are used in the clinic, so that vaccine makers, regulators, and doctors can learn from this article.
Nanovaccines
Nanotechnology is a modern way to make vaccines that are only given to certain people. NPs are any particles or groups of particles with sizes between 1 nm and 999 nm. They are used in nanotechnology. Nanovaccines can be made from metals, polymers, biological macromolecules, and other things. They are between 1 nm and 100 nm in size [5]. This size range is home to many disease-causing organisms, especially viruses, which shows that the immune system of the host has evolved to fight particles in this size range [6]. NPs can be made to interact with B-cells and/or phagocytic cells, which makes them an ideal platform for making vaccines [7]. Some nanovaccines, on the other hand, can have antigens or immune-stimulating molecules inside of them or built into the structure of the particle itself. Most nanovaccines are covered with antigens that are of interest, which lets them interact directly with B-cell receptors [8]. Antigen particle presentation has been shown to stimulate the immune system more than recombinant protein antigen alone. This implies that using a nanovaccine to produce immunogens may be advantageous [9]. NP-based vaccines are an alternative to traditional vaccines [10]. NP-based vaccines might have benefits like payloads, surface properties that can be changed, high sizes that can be changed, controllable drug release rates, and better stability. Aikins et al. [11] have made a lot of NP vaccine platforms with extra adjuvant properties and the ability to target antigen-presenting cells (APCs) to boost immunity.
Organic NPs
Organic NPs are made of organic molecules that can be found in nature or made in a lab. Organic NPs can be found in nature in many different forms, such as emulsions, lipid bodies, milk protein aggregates, and even more complicated structures like viruses. Most organic materials are biodegradable, nontoxic, and compatible with living things, while most inorganic materials are more stable, have smaller particles, can be tuned to specific needs, have high antigen loadings, are more permeable, and have a controlled release profile. Gregoriadis [12] said that many different organic NPs have been used as platforms for vaccines because they are biocompatible, biodegrade, and don’t cause much harm in general. Purely organic NP platforms have many advantages over other existing NP platforms, such as the ability of antigens and adjuvants to self-assemble in physiologically mild conditions and the ability to accommodate a wide range of compositions, sizes, surface modifications, and modalities [13]. There are recent changes to the organic NP vaccine delivery platform, such as virus-like particles (VLPs) and liposomes, which are polymeric NPs (PNPs).
NPs made of polymers
PNPs have become more common in vaccines over the past few years because their properties, such as composition, particle size, and surface charge, can be changed (Figure 1A). This makes it possible to create a platform for delivering vaccines with multiple functions to specific hosts [14] (Table 1). Polylactic acid (PLA), polyglycolic acid (PGA), and polylactic-co-glycolic acid (PLGA) are the biocompatible polymers used to create PNPs. Although it decomposes slowly, PLA is thick and bendable. But PGA is rigid but deteriorates rapidly after use. According to the research [15], PLGA shares characteristics with both PGA and PLA. This implies it can be tailored to incorporate desirable qualities from both polymers. PNPs can be made to serve as a depot for antigen release and exposure to APCs under physiological settings by modifying the co-polymer composition during production [16]. The recombinant SARS-CoV-2 spike protein subunit 1 (rS1) integrated in a more conserved E structure i.e. the rS1-E fusion protein (rS1-E) covered on PLGA forms the rS1-E-PLGA nanovaccine, which has a suitable size, shape, good stability, and sustained release [17]. The severe acute respiratory syndrome coronavirus 2 (SARS-CoV-2) rS1-E-PLGA nanovaccine was evaluated for its immune effect in BALB/c mice [18]. This is crucial for mucosal immunizations. In order to administer hepatitis B surface antigen via the pulmonary route, Poon et al. [13] synthesized NPs from PLA and PLGA in varying concentrations. Larger PLA particles were found in solutions containing more PLA. Because of their larger size, rat alveolar macrophages were able to consume them with greater efficiency than they would have if they had been smaller. As a result, more cytokines were produced. Furthermore, PLGA can be encapsulated in a lipid membrane to further stimulate the immune system. By enclosing the PLGA-conjugated malaria antigen, vivax malaria protein 1(VMP001), in a lipid membrane, Moon et al. [19] demonstrated that PLGA-lipid NPs induced stronger humoral responses in vivo with 10-fold less soluble protein vaccination dosage and maintained equivalent antibody titters for up to 180 days. Also, Ebola virus (EBOV) glycoprotein crosslinked to cationic lipid-hyaluronic acid [multilamellar vaccine particles (MVPs)] produced durable antigen-specific CD8+ and CD4+ T cell responses, and a single dose of MVP immunization protected 80% of mice from EBOV infection [20].
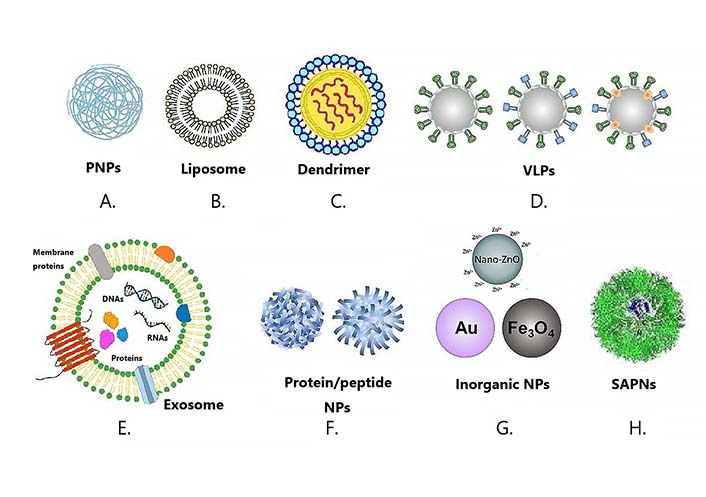
Various NP platforms for vaccine development. Types of nanovaccines: various NPs have been used for the development of nanovaccines. A. PNPs; B. liposome; C. dendrimer; D. VLPs; E. exosome; F. protein/peptide NPs; G. inorganic NPs; H. SAPNs. SAPNs: self-assembling protein NPs
Roles of different types of NPs in vaccine development
Types | Vaccine NPs | Roles in vaccine development | Disease cured | References |
---|---|---|---|---|
1. Organic NPs | PNPs | Better immunogenicity can be obtained by easy modification of surface proteins, biodegradable and targeted antigen delivery | Hepatitis B virus (HBV), malaria, Ebola, and Mycobacterium tuberculosis (M.tb) | [11, 20, 24, 26–28] |
Liposomes | Have the ability to protect against degradation, carry single or multiple hydrophilic and lipophilic antigen, control the release of antigen, enhance cellular uptake, and improve antigen- specific immune response | HBV, hepatitis A virus (HAV), human immunodeficiency virus (HIV), influenza A viruses (IAVs), and influenza B viruses (IBVs) | [29–32] | |
Dendrimers | Have adjuvant properties and provide molecularly defined multivalent scaffolds to produce highly defined conjugates with small molecule immunostimulators and/or antigens | Ebola; hemagglutinin type 1 and neuraminidase type 1-influenza A (H1N1); Toxoplasma gondii | [33–35] | |
VLPs | The particles mimic the parent pathogen, have high gastrointestinal stability, and possess self-adjuvant properties | Human papillomavirus (HPV), H1N1 IAV, HIV, H5N1 IAV | [36, 37] | |
2. Inorganic NPs | Gold NPs | Have the ability to tune and upregulate immune response, can exert optimal actions both as delivery systems and as adjuvants | West Nile virus, foot and mouth disease virus, H1N1 IAV, Streptococcus pneumonia, and Burkholderia mallei | [38–42] |
Iron oxides NPs (IONPs) | Promote the activation of immune cells and cytokine production, including potent humoral and cellular immune responses | M.tb, malaria, HBV | [43, 44] | |
3. Self-assembled NPs | Offer a collective strength of multiple binding sites and can provide improved antigen stability and immunogenicity | EBV, malaria | [45–47] | |
4. Protein/peptide NPs | Offer a collective strength of multiple binding sites (avidity) and can provide improved antigen stability and immunogenicity | HIV, influenza, and malaria | [45, 48] |
Chitin and chitosan are biopolymers with excellent bioactive properties, such as biodegradability, non-toxicity, biocompatibility, haemostatic activity, and antimicrobial activity [21]. Chitosan is harmless and biodegrades. Chitosan NPs can transfer antigens and immunoadjuvants to the mucosa when a vaccination is given [22]. The nasal and intestinal mucosa absorb PNPs better to boost immune response. The epithelium’s negatively charged cellular membrane interacts with positively charged chitosan-containing nanovesicles. Chitosan’s bioadhesivity prolongs the residence time of drug-loaded system and provides localized drug delivery [23]. Chitosan-based PNPs administer vaccines. Chitosan NPs provide T-cell epitopes of Esat-6 and fms-like tyrosine kinase 3 (FLT3) ligands against M.tb [24]. Chitosan NPs protected M.tb H37Rv-treated C57BL/6 mice. Interferon (IFN)- and interleukin-12 (IL-12) levels were 30–40% greater than the Esat-6/FL plasmid and 20% higher than the commercial tuberculosis vaccination [Bacillus Calmette-Guerin (BCG)]. Four weeks after the challenge, BCG and DNA plasmid-treated mice had approximately half the bacteria. Karayianni et al. [25] observed that the chitosan prevented nucleases from breaking down the DNA plasmid and helped cells enter the bloodstream, improving immunological response. Chitosan-PNPs can also prevent HBV infections. Karayianni et al. [25] created crosslinked chitosan polysaccharidic NPs to convey recombinant HBV antigens. These NPs produced 9 times more HBV-specific immunoglobulin G (IgG) than an aluminum-adsorbed vaccination in HBV-infected mice’s muscles. Block copolymers can be modified to improve PNP vaccinations against infectious illnesses.
Liposomes and lipid NPs
Liposomes are a special type of organic NPs that have been used in medicine more than any other NP platform [49]. These NPs are made up of biodegradable phospholipids that self-assemble into a lipid bilayer around an aqueous core when they get wet (Figure 1B). When a lipid bilayer is added to the surface of a particle, it can make the particle more stable. This is because liposomes are mostly impermeable to things like salts and macromolecules that are known to break down particles in biological conditions [50]. Because liposomes are so flexible, they can hold both hydrophobic and hydrophilic molecules, like antigenic proteins and peptides, in their lipid bilayer and aqueous core, respectively [51]. Several liposomal platforms have been approved for use as vaccines for infectious diseases. The EpaxalTM vaccine, which was made by Crucell Berna Biotech, was the first approved liposomal vaccine for treating HAV [29]. It had inactive virosomal hepatitis A vaccine (strain RG-SB) in it. Unlike traditional liposomes, the phospholipid bilayer of the Epaxal vaccine contained viral envelope (Env) glycoprotein. The lipid parts of the Epaxal vaccine, dioleoylphosphatidylethanolamine (DOPE) and 1,2-dioleoyl-sn-glycero-3-phosphocholine (DOPC), made it easier for immunocompetent cells to take in HAV antigens and kept the immune response from HAV injections going for more than 20 years after two booster doses. Crucell Berna Biotech made another liposomal particle called Inflexal®V vaccine, which was used to prevent flu by putting haemagglutinin from influenza A and B virus strains inside lecithin-phospholipid. Patois et al. [52] showed that the immunogenicity of the Inflexal®V vaccine is four times higher than that of the InfluvacTM vaccine.
Nanovaccines also use lipids (Figure 1B). Antigen-coated lipid-based NPs can behave like protein-based ones [53]. These antigens can be attached to the membrane using fatty acids, diacylglycerol, prenyl chains, or glycosylphosphatidylinositol (GPI). They are introduced directly to the membrane during liposome synthesis. Tags or chemical cross-linking to bilayer anchors can also add antigens [53]. Liposomes are lipid-based NPs with a phospholipid bilayer (unilamellar or multilamellar) around an aqueous core and antigens on the outside [54]. Liposomes carry medications and immunomodulatory substances in the aqueous core or membrane [55]. Army monophosphoryl lipid A (MPLA) head group and QS-21 carbohydrate boost immune cells in army liposome formulation with MPLA and QS-21 (ALFQ) [56] (Table 1).
Vaccines are carried by virosome liposomes. A virosome liposome has two phospholipid layers. Their membranes include viral glycoproteins. The virosome can target various cell types. An influenza-based virosome contains hemagglutinin, neuraminidase, and the target antigen. Like liposomes, virosome cores can include drugs or immunostimulants [57]. Lipid-based NPs can carry nucleic acid vaccinations (Figure 1B) [58, 59]. Lipid NPs (LNPs) [60] are like liposomes but can be single-layer micelles. Cationic or ionizable lipids protect LNPs nucleic acids from nucleases. LNPs can accelerate nucleic acid through the cell membrane and into the host cell depending on their lipids [60, 61].
There is a difference between liposomes and LNPs. Antigen coating on LNPs mainly improves the immunogenicity of the antigen. And the liposome is a micro vesicle. The drug that needs to be delivered is wrapped in liposome to better protect the stability of the drug. Therefore, LNPs mainly deliver substances with strong antigenicity (various immunogens), while liposomes focus on unstable substances (nucleic acid) [62]. LNPs simultaneously play a key role in the development of COVID-19 mRNA vaccines as vaccine carriers and adjuvants [63]. According to the World Health Organization (WHO), a total of 18 mRNA-based COVID-19 vaccine candidates have proceeded to clinical trials to date, most of which are based on ionizable LNPs [64, 65].
Dendrimers
Dendrimers are synthetic globular polymers that are well-defined (monodisperse) and have a wide range of interesting chemical and biological properties (Figure 1C and Table 1). Chemical properties include the presence of multiple functional groups on the surface that are easy to access and can be used to link molecules that are important to biology, as well as methods that make it possible to precisely change the properties of surface groups. Dendrimers are very biocompatible and their biodistribution and interactions with cell membranes can be predicted based on their size and surface charge. Dendrimers are very well-defined man-made macromolecules that have a high number of functional groups and a compact molecular structure. Dendrimers have the best properties to fill the need for effective immunostimulating compounds (adjuvants) that can make vaccines work better. Dendrimers can provide molecularly defined multivalent scaffolds to make highly defined conjugates with small molecule immunostimulators and/or antigens [33].
VLPs
Another way to get the immune system to respond more strongly is to make VLPs (Figure 1D), which are carriers that look like the virus and have the same structure and properties that make them immunogenic [16]. VLPs are NPs that are round and usually self-assemble to be 20–200 nm in diameter [66]. Most of the time, these particles are made from the proteins of bioengineered bacteria, yeast, insects, mammals, or plants [67]. VLPs are made of highly purified viral proteins that are free of their host’s genetic material and can be used to mimic parts of the original virus (Table 1). In particular, these particles can protect against a wide range of different viruses. VLPs are generally very stable at high temperatures and don’t need cold chains to stay alive. They can be used to vaccinate people in developing countries where cold chains are hard to come by [36]. The success of the Recombivax HB®, Gardasil®, and Cervarix® vaccines in the clinic [67] proves that VLPs are a good idea. The Recombivax vaccine was the first VLP to be approved by the Food and Drug Administration (FDA) to prevent HBV. This changed the way recombinant DNA is used to make vaccines for good. Soon after, the FDA approved the second and third nanoscale vaccine systems, Gardasil and Cervarix, which are currently used to protect against HPV.
Exosomes
Exosomes are nanovesicles that come from cells and help move things from one cell to another. Small molecules or drugs based on nucleic acids can be put into exosomes and then sent to specific types of cells or tissues. This is called “targeted drug delivery”. Exosomes, which are nanosized vesicles, are becoming popular as drug delivery systems for therapeutics because they come from nature, can help cells talk to each other, and can hold biological molecules like proteins and nucleic acids inside the lipid bilayer membrane or in the lumen (Figure 1E). Exosomes have proteins, lipids, and RNA that come from the body and could be used to deliver cargo to target cells. This could help diagnose and treat several diseases. Exosomes offer better delivery of cargoes in vivo because they can travel safely in extracellular fluid and get their cargoes to their target cells very well. But problems with stabilizing the exosomes, making enough exosomes in a safe and effective way, getting drugs into the exosomes efficiently, getting rid of the exosomes from the bloodstream, and moving from the lab scale to clinical production may slow down their development and use in the clinic. For the clinical use of exosomes, it is important to understand how exosome vesicles move and work at the molecular level [68].
Protein/peptide NPs
Nanovaccines can be made from any material that fits within the size range, but when it comes to developing vaccines, most attention has been paid to particles made from biological macromolecules [69]. Protein-based assemblies have dominated nanovaccine research because they are easy to work with and have many different secondary structures [70] (Figure 1F and Table 1). One way to do this is to use “virus-like particles” or VLPs (Figure 1F). These particles are made of virus capsid proteins that can self-assemble. They look like their parent viruses, but they don’t have any genetic material, so they can’t spread disease [71]. VLPs can be enveloped (eVLP) or not enveloped (non-eVLP), and they can be made from viral capsid proteins from different parents, modified viral capsid proteins, or a mosaic of multiple viral capsid proteins [72]. Protein-based NPs can also be made by using naturally occurring protein assemblies like ferritin, heat shock protein, or the E2 subunit of the pyruvate dehydrogenase (SAPNs, Figure 1F) [70]. These naturally occurring protein groups self-assemble into nanostructures. Using basic molecular biology techniques, antigens can be designed to be expressed directly on the protein or added after the nanostructure has formed [70].
Inorganic NPs
Most of the time, inorganic NPs have been used in cancer as contrast agents for imaging or for photothermal therapy. In preclinical settings, there has recently been a lot of interest in making inorganic NPs into vaccines. Most inorganic materials have smaller particles, better stability, controlled tunability, better permeability, high drug loadings, and a controlled release profile [73]. This makes them perfect for delivering antigens as a vaccine [13]. Most of these newer generations are made with an inorganic core and an organic shell, making them hybrid inorganic nanomaterials. These inorganic NPs are now being used in vaccines as both carriers and boosters (Table 1).
Gold NPs
Gold NPs (AuNPs) have been used in many different ways, such as in computers, catalysis, sensing probes, and drug delivery (Figure 1G) [74, 75]. AuNPs are perfect for use in vaccines because they aren’t too dangerous and can be made in different ways to have different sizes, shapes, and chemical groups on their surfaces. The Turkevich-Frens method, which uses citrate ions as both a reducing agent and a capping reagent to make spherical AuNPs with a diameter of 10–20 nm [76], is used to make AuNPs in situ. Recent synthesis methods, like seed-growth methods, make it easier to control the size and shape of NPs by changing the reducing agent, temperature, pH, solvent, and synthesis time [74] (Table 1). This makes it possible to make rod- or cube-shaped NPs that are 2–150 nm in size. The way the host cells take in and fight off the virus depends on its shape and size. Niikura et al. [38] looked at how spherical, rod-shaped, and cube-shaped AuNPs coated with West Nile virus Env protein affected the immune response. It was found that macrophages and dendritic cells (DCs) take up rod-shaped AuNPs better than spherical or cube-shaped AuNPs. But spherical and cubic AuNPs caused more inflammatory cytokines like tumour necrosis factor (TNF)-, IL-6, IL-12, and granulocyte-macrophage colony-stimulating factor (GM-CSF) to be released, while rod-shaped AuNPs caused more inflammasome-related cytokines like IL-1 and IL-18 to be released. So, antibody production doesn’t depend on how well the AuNPs are taken up, and different shapes of AuNPs could boost immune responses in different ways. Chen et al. [39] also looked at different sizes of spherical AuNPs with diameters from 2nm to 50 nm that were linked to a synthetic foot-and-mouth disease virus peptide. Since peptides can be put on the outside of a NP, vaccines made with peptide-based NPs can get into cells better than those made with whole proteins. When compared to the control, a synthetic peptide conjugate, they found that antibodies responded best to AuNPs with a diameter of 8–17 nm.
Tao and Gill [40] showed that AuNPs functionalized with the extracellular domain of M2 peptide [matrix protein 2 ectodomain (M2e)] can protect against influenza A. In addition, soluble cytosine-phosphorothioate-guanine oligodeoxynucleotide (CpG ODN) was added to the AuNP to stimulate Toll-like receptor-9 (TLR-9), which was important to protect against influenza pneumonia. When soluble M2e peptide was added to the AuNP formulation, mice that were infected with the H1N1 flu virus had a high level of M2e-specific IgG antibody, which completely protected them.
IONPs
IONPs are most often associated with magnetic resonance imaging (MRI) contrast agents, which have been used to image a wide range of diseases [77] (Figure 1G and Table 1).
In a study, Ruiz-de-Angulo et al. [78] showed that engineered IONPs can become a cornerstone for enhancing immunotherapy owing to the coupling of catalytic activity with immunomodulation. In 1996, the FDA approved their use in clinical settings. Recently, efforts have been made to use IONPs as adjuvants in the development of vaccines. Iron is an important part of what starts the inflammatory process. Rojas et al. [79] used murine M2 macrophage models to make superparamagnetic IONPs (SPIONPs) that were coated with dimercaptosuccinic acid (DMSA), aminopropyl silane, or aminodextran. The researchers found that treating M2 macrophages with SPIONPs caused reactive oxygen species to be made, changed how iron was used in the body, and helped regulate the immune system by making IL-10. Shen et al. [80] did a study in which SPIONPs were given intravenously to mice that had been sensitized to ovalbumin (OVA). This showed that this was true. The decrease in OVA-specific IgG1 and IgG2a antibodies shows that these SPIONPs cut down on T cell-mediated immune reactions. These SPIONPs also decreased the production of cytokines by splenocytes that were re-stimulated by OVA antigens. Because of these things, IONPs showed a lot of promise as a platform for making vaccines against infectious diseases. Iron oxide and iron oxyhydroxide NPs (IOHNPs) impaired SARS-CoV-2 infection of cultured cells [81]. The study suggests that IONPs and IOHNPs may be repurposed to be used as prophylactic or therapeutic treatments in order to combat SARS-CoV-2 infection [81].
ZnO-NPs
The FDA has said that Zinc oxide NPs (ZnO-NPs) are “Generally Recognized as Safe (GRAS)” and that they are an effective anticancer drug [82]. Due to their low toxicity, ZnO-NPs can be used in a variety of biological ways, such as being an antioxidant, antibacterial, antifungal, anticancer, and anti-diabetes agent (Figure 1G). They can also be used in agriculture [2, 83].
SAPNs
Self-assembling NP vaccines with the receptor-binding domain (RBD) of SARS-CoV-2 were made (Figure 1H and Table 1). The vaccine candidate elicited strong humoral and T-cell responses and protected rhesus monkeys from infection by 2 105 CCID50 SARS-CoV-2 with short-term shedding and only minor pathological damage. Protein complexes that have been cleverly assembled can be used to produce vaccines [69]. Protein complexes of the size and form of a little virus are synthesized from scratch [5]. All the advantages of current protein nanovaccines are present in these nanoassemblies, with the added bonus of greater control over antigen shape and dosing thanks to their in-house fabrication [69]. One application of this science is in SAPNs. Proteins arrange themselves into complex three-dimensional structures in water (Figure 1H) [6]. In malaria, influenza, and toxoplasmosis animal models, SAPNs have been demonstrated to be immunogenic and protective [7, 8].
In the 1950s, a protein extracted from the tobacco mosaic virus (TMV) was found to form rod-shaped particles that looked like the original TMV but didn’t have any genetic material [45]. This was one of the first examples of a self-associating protein particle. Later, in the 1970s, the hepatitis B virus surface antigen (HBsAg) was separated from infected human serum [45]. For example, almost all living things make ferritin, which is a protein whose main job is to store iron inside cells. Ferritin is made up of 24 subunits, each of which is a bundle of four alpha-helices. These subunits come together on their own to form a quaternary structure with octahedral symmetry. Several high-resolution structures of ferritin have been found, and they show that Helicobacter pylori ferritin is made up of 24 identical protomers. In animals, however, there are both light and heavy chains of ferritin, which can form particles of 24 subunits on their own or in different proportions.
Ferritin
Ferritin, which is made by most living things, is an interesting self-assembling NP-based vaccine platform for infectious diseases [84]. Ferritin is made up of 24 alpha helix subunits with 3-fold axis symmetry. These subunits come together on their own to form NPs that are more stable thermally and chemically [85]. The shape of ferritin NPs makes it possible for trimeric antigens to be shown in a good way, which increases the chance that they will protect against different subtypes of influenza virus.
Discussion
The immune system and nanovaccines
Nanovaccines that are meant to cause humoral immune responses to put a lot of focus on exposing Env. Different candidates have gone after either the precursor protein gp160, one or more of the processed sections of Env (gp140, gp120, or gp41), or certain parts of Env, like the variable loops or membrane-proximal external regions (MPERs) [84–88]. By putting Env or one of its many subunits on NPs, many of the factors that are thought to be important for HIV-1 immunogen formation can be incorporated into the design, which could lead to better responses. NP immunogens can also carry Env trimers, just like natural Env trimers do. Stabilized envelope glycoprotein (Env) (SOSIP trimers) are native-like structures made from gp140 and disulfide bridges that lock the structure into a native-like prefusion conformation. These structures were used to build a lot of Env [88–92]. In addition to being on the surface of NPs [86, 87, 93–95], these proteins can cause functional responses in animals that have been immunized [86, 87, 93]. Also, nanovaccines can be made to show the Env protein or its parts in a shape similar to their natural form without adding more disulfide bridges [84, 96].
NPs could be used to fix the problem that there aren’t enough Env molecules on the surface of the virus. Different nanovaccines can be made with different symmetries and shapes that make it easier to show off Env or a specific part of Env. Antigens can also be put at a distance of 5–10 nm, which is the best distance for activating B cells [97–99]. This change allows for better cross-linking of the B-cell receptor, which leads to better immune responses. With this change, you can make antibodies that have higher titers than recombinant proteins or even the HIV-1 virus itself.
Nanovaccines and neutralization
Nanovaccine research for HIV-1-specific antibodies focuses on nabs [100]. VLPs from HIV-1 have been attempted. These vaccinations can induce Tier-2 neutralizing antibodies (NAbs), but they have many of the same issues as the HIV-1 virus, including low Env presentation, unstable and incomplete spikes, and longer spike intervals [101, 102]. Other attempts to make fusion VLPs by combining HIV-1 group specific antigen (Gag) or capsid proteins from other viruses with HIV-1 proteins like gp160, gp120, trimeric gp140, third hypervariable loop (V3 loop), first and second hypervariable regions (V1V2 loop), and CD4 binding site yielded mixed results, with only a few making NAbs in animal models [103–112]. Animals administered a Gag-VLP with MPER made few gp41-specific antibodies to inhibit the virus. However, animal models responded less to gp41 on non-eVLPs from other viruses [113, 114].
Ferritin NPs are naturally occurring iron-binding nanocages consisting of 24 monomers that self-assemble [100, 115]. SOSIP on ferritin NPs is more immunogenic than soluble SOSIP, although it didn’t aid neutralization significantly [92, 116, 117]. SOSIP’s design or the ferritin nanovaccine’s eight timers may explain this [100]. Sixty Aquifex aeolicus lumazine synthase monomers form a NP with 20 trimers [118]. The Env outer domain (eOD) of gp120 was attached to these particles to activate VRC01-type broadly NAb (bNAb) germ-line progenitors [119]. After vaccination, transgenic mice produced broad-NAbs. This candidate is awaiting Phase I clinical trials [100, 120].
Liposomes, like protein NPs, help create NAbs [121]. Most nanovaccine research has focused on how to deliver peptides or proteins to the host immune system to make them immunogenic [100]. Antibodies targeting gp41’s MPER are a priority. Infected patients have bNAbs that bind to the protein and viral Env [122–124]. Liposomes coated with the entire gp41 subunit or MPER peptides increased antibody titers. This may cause weak to moderate neutralization and cross-reactivity [85, 125, 126]. Virosomes delivered gp41 as part of a mucosal vaccination technique that caused NAbs to develop in the vagina of vaccinated nonhuman primates (NHPs) and protected most animals when challenged [127]. Most Phase I trial participants had mucosal antibodies [128].
Liposomes deliver Env trimers. SOSIPs on liposomes have been attempted in several articles [86, 129]. As expected, the liposomal virus rendered it immunogenic and harder to fight [86, 129]. Liposome-based nanovaccines were created to circumvent the glycan barrier [129]. Changes in glycans and a prime-boost technique focused the immune response on the CD4 binding site. Cross- NAbs and a bNAb that bound to a novel glycan/protein epitope resulted [129].
mRNA vaccines in lipid NPs are a promising area of vaccine research [130]. Two research examined HIV-1-neutralizing antibody production using Env-coding mRNA vaccines [131, 132]. In the first investigation, rabbits and NHPs made NAbs, and NHPs made tier 2 NAbs [131]. These results were expanded to show that nucleoside-modified mRNA generated NAbs that lasted at least 41 weeks to a protein-based immunogen with the identical sequence [132].
Nanovaccines and non-NAbs
Human clinical investigations have only connected non-NAbs to protection (RV144, the Army-led Thai HIV vaccine efficacy trial). Despite the fact that most studies investigating the generation of humoral responses in response to a nanovaccine have focused on NAbs, it is the non-NAbs that have been demonstrated to correlate with protection. Antibody-dependent cell-mediated cytotoxicity (ADCC), antibody-dependent cellular phagocytosis (ADCP), antibody-dependent cell-mediated virus inhibition (ADCVI), and antibody-dependent complement activation (ADCA) all result from the Fc portion of the manufactured antibody binding to the Fc receptor on effector cells. ADCC was observed in those who had a lower susceptibility to the RV144 virus. It’s likely that the significance of these reactions was underestimated or under-researched due to the complexity and inconsistency of the assays utilized for quantification [133, 134]. Some antibodies defy easy categorization as neutralizing or non-neutralizing. Instead, they can pursue multiple potential solutions simultaneously [135–139]. In the passive transfer of previously discovered neutralizing monoclonal antibodies (mAbs) into NHP models, non-neutralizing responses have been linked to 25–45% of the antiviral activity [140–142]. This demonstrates how critical it is for the advancement of vaccinations.
When investigating the potential for a nanovaccine to have an effect other than neutralization, one of the earliest experiments focused on a VLP containing various fragments of gp41 [113]. In contrast to VLPs without the HR2 region, those containing it were able to activate ADCC [113]. In a second prime-boost vaccine research, VLPs were utilized in conjunction with two DNA injections and two Gag-VLP injections. Mice that received these injections displayed both ADCC and ADCIV activity [143]. Animals that had been injected with a SAPN depicting the V1V2 loop of Env displayed ADCP activity in another investigation [96]. Vaginal ADCC-inducing antibodies were produced after a mucosal vaccination in NHPs [57]. After receiving LNPs-encased mRNA vaccinations, both rabbits and nonhuman primates developed antibodies with the potential to trigger ADCC [131]. Additionally, mRNA analysis revealed that vaccinated NHPs produce antibodies with the potential to induce ADCC and ADCP activity [132].
Nanovaccines and cell-based immunity VLPs were used in some of the earliest attempts to create vaccinations that would elicit CD4+ or CD8+ responses [144]. Making Gag NPs, with or without additional antigens like Env, was a major focus of the earliest studies. Cytotoxic T lymphocytes (CTL), T helper (Th) cells, and antibody titers were all found to increase, however, the exact amounts varied between trials and between related antigens [107, 109, 110]. More plans were devised to boost these figures. In the first strategy, CD40 ligand (CD40L) was included in the Gag-VLP to bind to CD40 and activate DCs, hence increasing the virus’ immunogenicity [145, 146] When mice were administered VLPs containing CD40L, their CD4+ and CD8+ cell responses to p24 were significantly higher than when mice were given VLPs alone [145]. NHPs exhibited enhanced cellular and humoral immune responses after receiving a simian HIV (SHIV) Gag-VLP including Env and CD40L as a vaccine [147]. Similar VLPs including Env and CD40L were employed in a simian immunodeficiency virus (SIV) challenge model. Anti-Env antibodies, CD4+ and CD8+ responses rose after vaccination, although infection was still transmitted. Mice that were administered SHIV Gag-VLP along with CD40L showed significantly reduced viremia [147].
The primary function of VLPs in T-cell-based vaccines has been to serve as the “boost” component of the “prime-boost” strategy [17, 148–151]. One experiment used a DNA prime together with a Gag-VLP displaying reverse transcriptase or a Tat: Nef fusion protein. VLP was more effective than two DNA-only vaccines at boosting CD4+ and CD8+ responses [151]. The CD4+ and CD8+ immunological responses of mice were enhanced when they were first given two doses of a DNA vaccination, and then two doses of a Gag-VLP vaccine [17, 143]. The CD4+ and CD8+ responses of mice immunized with DNA were lower than those of mice immunized with a VLP displaying gp120 and a rMVA (recombinant modified vaccinia Ankara viruses) vector, as demonstrated in another research [148]. This demonstrates the critical role of prime-boost in the formation of effective immune responses against HIV-1 [148].
Future prospects
Making a nanovaccine effective enough to minimise sickness and death worldwide has been and continues to be a primary objective of the scientific community. Various approaches have been tried to determine which immunogen could be most effective. This conversation has been more challenging due to several factors, including the vast diversity of viruses, the lack of information about which immune response is most crucial for preventing infection or eliminating the virus, and the absence of a reliable animal model that accurately mimics HIV-1 infection. Using what we’ve learned over the previous almost 40 years, we’ve developed strategies that may result in a more robust immune response, such as native-like trimers, mosaic sequences, and prime-boost vaccine schedules.
Historically and prospectively, nanotechnology’s primary contribution to vaccine development has been as a vehicle for administering the vaccine. Nanovaccines have been proven to elicit better immune responses than soluble protein alone in numerous preclinical and clinical testing. Even if this strategy has been applied to HIV-1 research, there is still a long way to go. Modern antigen production methods, such as the native-like SOSIPs and mosaic vaccines, can and have been implemented using a variety of NPs. Depending on the particle's size, shape, or chemical composition, nanotechnology can be used to establish a direct connection with specific types of cells. It is possible to create nanovaccines that transport targeted epitopes in a form mimicking endogenous tissue. Thus, the immune system is freed up to respond more effectively to actual threats by reducing responses that aren’t necessary or helpful. Nanovaccines will play an increasingly important part in their development as technology advances.
Nanovaccines are a broad and complex topic with many subfields. The ideal antigen or immune response cannot be achieved with a single NP. There are a lot of factors to consider while deciding how to manufacture nanovaccines. To think of it as simply attaching an antigen to a pre-existing NP is to miss the point of this dynamic process. Antigen, carrier, adjuvant, and vaccine formulation are all optimized throughout the course of a series of steps. Biophysical tuning of the NP and antigen(s) is required to ensure that the antigen is displayed accurately, that the ideal density and distance are attained, and that the formulation is stable and immune stimulating. A successful nanovaccine formulation is impossible without first resolving all of these concerns. Multiple examples suggest that prime-boost techniques may be the wave of the future for nanovaccines. Because of this, nanovaccines can play a crucial role as either the “prime” or the “boost”. Making antibodies targeting a specific region requires first priming the immune system with a larger antigen, such as a SOSIP on a NP, and then boosting with parts of the antigen, such as the native-like presentation of the V2 loop, on a well-crafted NP. This method can also be used to produce a more effective vaccination against HIV-1 by using boosts from many HIV-1 strains.
It is expected that within the next five years, a significant number of HIV-1 vaccines will be manufactured using nanovaccines. There are still many avenues open for exploring and employing nanovaccine technology, in addition to exploring and employing new antigens and methods of delivering vaccinations. It’s evident that vaccinology has entered a new era with the advent of mRNA vaccines. NPs are essential for administering mRNA vaccines and their use is expected to increase in the next years. Although mRNA vaccines have been demonstrated to be effective against COVID-19, it is unclear how long the responses will last. An enhanced immune response may result from combining an mRNA vaccination with a protein boost supplied by NPs. It’s possible that this trend is being observed in the HIV-1 field, where the ever-evolving virus is met with responses based on a variety of antigens.
Antigen cross-presentation is used to make an anti-cancer nanovaccine
Antigen cross-presentation is the process by which DCs put antigens from outside the body on major histocompatibility complex (MHC) class I molecules. This activates CD8+ T cells and helps get rid of tumours. DC-mediated antigen cross-presentation is controlled by a number of things, such as the stage of maturation of DCs, co-stimulatory signals, the microenvironment of T-cells, the way antigens are taken into cells, and adjuvants. Recently, the ability of DCs to cross-present tumour antigens may have led to the success of new cancer immunotherapies. Warrier et al. [152] reported recent changes in making new nanovaccines for strong CD8+ T-cell responses in cancer. In this context, recent studies have been focusing on nanocarriers that have been used as antigen cross-presentation-based cancer immunotherapies.
STING-activated cancer immunotherapy nanovaccine
The first step in a cancer-specific, cellular immune response is to have antigen presented to T cells by the most potent APCs—DCs [153]. One of the hardest components of creating a robust T-cell response is spatial and temporal coordination of antigen cross-presentation in APCs after innate activation. Luo et al. [154] create a simple nanovaccine from an antigen and PC7A, a synthetic PNP. Despite little systemic cytokine response, this nanovaccine induces a robust cytotoxic T-cell immune response. PC7A NP transfers tumour antigens from the cytosol to lymph node-draining APCs. Type I IFN-activated genes activate surface presentation. This impact is caused by the stimulator of IFN genes (STING), not the Toll-like receptors (TLR) or mitochondrial antiviral-signaling (MAVS) pathway [154].
In melanoma, colon cancer, and HPV-E6/E7 tumour models, nanovaccine inhibited tumour growth significantly. In a TC-1 tumour model, combining a PC7A nanovaccine with an anti-PD-1 antibody resulted in 100% survival after 60 days. Rechallenging these tumour-free animals with TC-1 cells completely inhibited tumour growth, implying the formation of long-term anticancer memory [154].
Lymphoid nanovaccines boost cancer immunotherapy
More studies into cancer therapeutic nanovaccines demonstrate that poor delivery to lymphoid organs causes the immune system to respond slowly to tumours. The rapid growth of tumours demands an effective mechanism, which distributes nanovaccines to lymphoid organs to immediately trigger an immune response. Traditional preventive vaccinations usually form a depot at the injection site to gradually trigger a long-lasting immune response. Optimizing nanovaccine size, shape, charge, colloidal stability, and surface ligands can increase lymphoid organ uptake. Dynamic nanovaccines can precisely target lymphoid tissues. Cai et al. [155] announced nanovaccine delivery strategy progress. It discusses future difficulties and plans [155].
Systems with NPs for a cancer vaccine
Vaccines are good for public health because they keep people from getting sick by getting the body’s adaptive and innate immune systems ready to fight infection. There is growing interest in priming the host immune system for a thorough and focused antitumor response as we understand more about cancer and how it affects the immune system. NP systems have shown promise as ways to effectively deliver antigens as vaccines or boost immune responses as adjuvants. Several studies have attempted to address the critical physiological processes involved in vaccine delivery, current breakthroughs in the use of NP systems for vaccinations, and the issues that must be resolved before NP-based vaccines and adjuvants may be utilized by the general public [156].
Peptide-mediated nanovaccine delivery to cells
Small antigens, like proteins, peptides, or nucleic acids, are used in vaccinations to wake up the immune system and get it to respond in a way that protects against a pathogen. Instead of regular vaccines, scientists are working on nanovaccines right now. Nanovaccines are between 10nm and 500 nm in size, which makes them easy for cells to take up and gives them a better safety profile. But low-level immune responses, such as the removal of redundant pathogens, activate the immune system in a way that isn’t helpful and can be hard for APCs to recognize and take up (APCs). Also, toxicity can be a big problem. Cell-penetrating peptide (CPP)-mediated vaccine delivery systems based on nanotechnology have been proposed to solve these problems. Most of these systems are meant to improve the stability of antigens in vivo and their delivery into immune cells. Antigen delivery systems that include CPPs are very appealing. So, the unique ability of CPPs to translocate makes them an attractive carrier that can deliver cargoes in an efficient way. This makes them useful for delivering drugs, genes, proteins, and DNA/RNA vaccines. CPP-mediated nanovaccines can make it easier for APCs to take in antigen, process it, and show it to the immune system. These are the main steps that start an immune response. This article looks at the different ways CPP-based nanovaccines can be given to people [157].
Biotin-avidin-based virus-like nanovaccine for tumour immunotherapy
Viruses, microscopic disease-causing agents, are primarily proteins and nucleic acids. Long-term selection has improved animals’ viral defenses. The immune system can recognize viral proteins and sequence-specific nucleic acids like CpG ODN, single- and double-strand RNA, making virus removal easier. Researchers aimed to develop a cancer-fighting nanovaccine. Immunology inspired this notion. This nanovaccine is mostly made of nucleic acids (CpG ODN), proteins [including tumour-associated antigen and neutravidin (nAvidin) as skeleton materials for building nanovaccines and carriers for loading tumour-associated antigen and CpG ODN], and dye molecules for assembling nAvidin into virus-sized NPs and tracking the vaccine in vitro and in vivo. In vitro, the nanovaccine stimulates DC maturation, antigen cross-presentation, and cytokine production. In vivo, it targets lymph nodes, offers antigens to produce tumour-specific CD8+ T cells, and induces a Th1-biased immune response. Most crucially, this virus-like nanovaccine reduces antigen-expressed tumour growth and helps mice with tumours live longer [158].
Nanotechnology: morality and possible dangers
Larrouturou [159] says that having a sense of ethics is what makes us openly argue about the moral basis of our findings, turning the scientist back into a concerned citizen. In nanoscience, the idea of conquering complexity at a microscopic scale is sometimes used to scare people. Even if their concerns aren’t valid, scientists have a moral duty to talk about ethics in public. The first step in this argument is to admit that there is a huge gap between what is known about a few nanometric functions and what is known about life as a whole.
Conclusions
Nanovaccine needs viral vaccine research. Nanovaccines can deliver natural antigens and are immunogenic. Nanovaccines may aid HIV-1 vaccine development. After years, nanovaccine manufacture remains problematic. Immunization induces antibody and cell-mediated HIV-1 responses. A vaccine that works will likely cause both reactions. Nanotechnology can create nanovaccine immunogens. Lipid NPs, protein assemblies, VLPs, and liposomes are nanovaccines. Nanovaccines can have different surfaces or immune-stimulating antigens. These adjustments improve vaccination over soluble antigens. Preclinical nanovaccine technologies have produced effective vaccinations. Potential nanovaccines produce NAbs, CD8+, and CD4+ immunological responses. They are immunogens and “prime-boost” vaccinations. Nanovaccines may be more essential than thought in vaccine production. Infectious illness vaccines struggle to deliver antigens to APCs for long-term immunity. NPs improved vaccine delivery recently. Due to their properties, organic and inorganic NPs can carry vaccines. NPs delay antigen release. They reduce body antigen exposure. New clinical NP vaccinations could bring nanomedicine to the clinic. It is difficult to produce consistent, reproducible nanoparticles at a cheap cost using high-throughput and scale-up synthesis methods [160]. NP vaccines are harder to use and make. NPs can deliver vaccines clinically. The characteristic of nanovaccine is that it can modify the entire nano-delivery system according to the needs of disease treatment itself. It meets the needs of vaccine developers through the modification and combination of NPs.
Abbreviations
ADCC: |
antibody-dependent cell-mediated cytotoxicity |
ADCP: |
antibody-dependent cellular phagocytosis |
APCs: |
antigen-presenting cells |
AuNPs: |
gold nanoparticles |
CD40L: |
CD40 ligand |
CpG ODN: |
cytosine-phosphorothioate-guanine oligodeoxynucleotide |
CPP: |
cell-penetrating peptide |
DCs: |
dendritic cells |
Env: |
envelope |
Gag: |
group specific antigen |
H1N1: |
hemagglutinin type 1 and neuraminidase type 1-influenza A |
HAV: |
hepatitis A virus |
HBV: |
hepatitis B virus |
HPV: |
human papillomavirus |
IFN: |
interferon |
IgG: |
immunoglobulin G |
IONPs: |
iron oxide nanoparticles |
LNPs: |
lipid nanoparticles |
M2e: |
matrix protein 2 ectodomain |
NHPs: |
nonhuman primates |
NP: |
nanoparticle |
ODN: |
oligodeoxynucleotide |
OVA: |
ovalbumin |
PNPs: |
polymeric NPs |
SARS-CoV-2: |
severe acute respiratory syndrome coronavirus 2 |
SPIONPs: |
superparamagnetic IONPs |
VLPs: |
virus-like particles |
Declarations
Acknowledgment
The authors are thankful to the faculty members and researchers of Darrang College and Rajiv Gandhi University for their encouragement and support.
Author contributions
CB: Conceptualization, Formal analysis, Writing—original draft, Writing—review & editing. Papari Devi: Conceptualization, Writing—review & editing. Pankaj Das: Formal analysis, Writing—review & editing. PMS, BD: Validation and Writing—review & editing.
Conflicts of interest
The authors declare that they have no conflicts of interest.
Ethical approval
Not applicable.
Consent to participate
Informed consent to participate in the study was obtained from all participants.
Consent to publication
Not applicable.
Availability of data and materials
Not applicable.
Funding
Not applicable.
Copyright
© The Author(s) 2023.