Abstract
Interest in the mechanisms of aging of the immune system has not faded over the past 100 years, and it is caused by the immune-mediated development of age-related pathology, including autoimmune organ damage, reduced vaccination efficiency, atherosclerosis, the development of cardiovascular pathology, etc. In contrast to many other organs and systems, the immune system aging begins at an early age and has more pronounced changes that lead to the development of secondary pathology, which significantly affects life expectancy. But an effective strategy to restore immune function has not been developed yet. During this time, the mechanisms of age-related dysfunction of organs and cells of both the adaptive and innate immune systems were studied in detail—thymus involution, a decrease in the potential of hematopoietic stem cells, impaired differentiation and functions of immunocompetent cells, as well as the ways of their interaction. Numerous potential therapeutic targets have been identified and various approaches have been used to implement such therapeutic interventions. The review is devoted to replacement therapy using transplantation of hematopoietic stem cells (HSCs) and young lymphoid cells and tissues, cellular and systemic factor exchange in heterochronic parabiosis, and some other widely used life extension approaches. It has been proven that cell therapy using young cells to rejuvenate the old immune system, unfortunately, often turns out to be ineffective because it does not eliminate the root cause of age-related changes. The phenomenon of inflamm-aging that develops with age can significantly affect both the aging of the organism in general and the functioning of immunocompetent cells in particular. Therefore, the most promising direction in the restoration of immune functions during aging is systemic approaches that have a complex effect on the organism as a whole and can slow down the aging process.
Keywords
Aging, immune system, rejuvenationIntroduction
Historically, research in the field of immunology was initiated by Elie Mechnikoff, who received the Nobel Prize in 1908 for the discovery of phagocytosis. His authorship also belongs to the term “gerontology”, published in “Etudes on the Nature of Man” [1]. Through contributions to these studies, Metchnikoff is regarded as the founder of the field of the immunology of aging [2], which was subsequently developed and argued by Walford RL [3]. And over the past 120 years since the discovery of phagocytosis, the functions of the immune system and its age-related changes have been well studied, and published in a large number of publications.
The immune system has a multilevel structure, and most of the interactions between individual organs, lymphocytes, and tissues of the body are well understood due to the ability of hematopoietic stem cells (HSCs) and lymphoid cells to migrate with the bloodstream and be maintained in vitro. The decline in immune functions with age is also a well-documented fact, indicating the presence of disturbances in both native and adaptive immune systems. It has been established that the main age-related changes in the immune system are associated with a decrease in the functional activity of T-cells [4, 5]. This disorder may be due to many factors: thymus atrophy [6], a compensatory increase in homeostatic differentiation of T cells at the skin and intestinal mucosa [7–9], a decrease in the number of circulating naive T cells, and an increase in the number of memory T cells [10], as well as impaired function of innate immunity cells—natural killer (NK) cells [11], macrophages, dendritic cells, as well as the interaction between them [12, 13]. All these changes lead to an increase in susceptibility to infectious diseases, an increase in the incidence of tumors, and pathologies which are associated with an increase in the frequency of autoimmune processes, and metabolic disorders caused by inflamm-aging [14, 15].
This review discusses advances in cell therapy using HSCs, heterochronic lymphoid organ transplantation, and young blood transfusion. The anti-aging cell therapy used leads to some of the positive changes seen in the aging immune system, but they are short-term and they do not affect life expectancy. The therapeutic use of systemic approaches associated with slowing down the aging of the organism as a whole and their effect on the immune system are also considered.
Ontogeny of the immune system
Embryonic period of immune system development
Like many other organs and systems, the development of the immune system is closely associated with the formation of other organs and systems of the organism, and it is aimed at creating effective protection against local damaging factors. The results of most studies indicate that the formation of the immune system occurs before the onset of puberty, and then progressive age-related changes begin to appear, resulting in manifestations of late-age immunodeficiency.
The formation of organs, including the immune system, begins in embryogenesis, and at birth, we already have a practically formed body defense system. It is known that in the early stages of ontogeny hematopoiesis in vertebrates occurs in three successive stages, in three different areas of the fetus, and allows the maintenance of erythropoiesis during the embryonic period, as well as the formation of bone marrow niches. The first stage of hematopoiesis begins in the yolk sac, resulting in the production of only three types of blood cells—primitive erythrocytes, megakaryocytes, and macrophages [16–19]. During the second stage, erythromyeloid progenitor cells are formed in the blood islands of the yolk sac, and during the third—HSCs are formed in the aorta-gonad-mesonephros area as a result of endothelial-to-hematopoietic transition [20, 21]. The formed progenitor cell lines of erythro-myeloid progenitors (EMPs) and HSCs migrate to the fetal liver subsequently, where they continue to differentiate with the formation of blood cells until birth [18]. As for the origin of mesenchymal stem cells (MSCs), which form the microenvironment for HSCs at birth, it still has controversial points. It was shown that in the bone marrow of the mouse embryo there are at least two populations of MSCs with different levels of nestin expression. One originates from the mesoderm, which does not express nestin, is characterized by intensive proliferation, and is involved in the process of bone tissue formation in the embryonic period. The second one originates from neural crest cells that express nestin and remains inactive during bone formation [22, 23]. Thus, by the time of birth, the microenvironment for HSCs in bone marrow can be formed by cells that belong to different germ layers during ontogenesis and, as a result, play different functions in the adult organism [21]. Thus, a complete hematopoietic system is formed in the bone marrow by the time of birth.
Unlike HSCs, the main task of which is the formation of progenitor cells of the hematopoietic system throughout the life of the organism, the functioning of the main players of the adaptive immune system—T- and B-lymphocytes—largely depends on the repertoire of receptors, which is usually accountable for antigen recognition, and the formation of which begins in embryogenesis. It is known that the development of B-cell receptor (BCR) and T-cell receptor (TCR) repertoires is strictly regulated during both ontogeny and during the formation of lymphocyte subpopulations after birth [24, 25]. In humans, prothymocytes with rearranged TCR genes appear as early as the 7th week of pregnancy in the yolk sac and liver of the embryo, while the entire gene loci of the alpha, beta, gamma, and delta TCR chains are available in the second trimester of the fetus [26]. Formation of the thymocyte repertoire is associated with the timing of thymic organogenesis, which begins in humans in the first trimester, and the thymus primordium is colonized by hematopoietic progenitor cells [27]. Similar dynamics are observed in the development of other species, with the diversity of the TCR γδ thymocyte repertoire reaching adult levels before birth [28, 29]. It should be noted that the proportion of γδ-thymocytes remains relatively low compared to their conventional αβ counterparts, whose differentiation in the thymus increases dramatically in the last trimester, and remains high after birth [29]. In general, TCR repertoires are functionally more similar in neonatal cord blood, but differ later in life as environmental exposure and ectopic antigens emerge [30, 31].
Like T cells, the development of different subpopulations of B lymphocytes also changes during life. The B-cell compartment in early ontogenesis is dominated by the formation of B-1 B cells, an innate subpopulation that can produce low-affinity immunoglobulin M (IgM) and provides a certain level of immune protection in the acute period [32]. B-1 B cells develop predominantly in the fetal and neonatal liver and then self-renew in adulthood [33, 34]. In this respect, B-1 cells differ from the normal follicular and marginal zone (MZ) subsets of B-2 cells, which are constantly differentiated from adult bone marrow progenitor cells [35].
Thus, at the stage of embryogenesis, the niches and repertoire of the TCR γδ thymocyte in the thymus, B-1 cell repertoire, as well as the relocation of HSC, and B cell development from liver to bone marrow (BM) are formed. At the same time, most of the TCR and BCR repertoire remains unchanged throughout the life of the organism.
Postnatal immune system development
Immediately after birth, a newborn’s body is exposed to multiple environmental factors—food components, allergens, and a rapidly developing gut, skin, lungs, and other mucosal microbiota. It has been shown that changes in the gut microbial population (e.g., due to antibiotic use) can have negative consequences for the postnatal development of the immune system, including increased susceptibility to autoimmune diseases and allergies [36, 37]. The mechanism of such interaction is extremely complicated. The results of a large number of studies demonstrate that the interaction of the immune system with the mucosal microbiome in the postnatal period critically affects the body’s susceptibility to the development of a number of immune-mediated pathologies in later life [38]. Activation of the neonatal immune system by microbiome cells has been shown to influence immune cell recruitment and function, as well as susceptibility to allergic and autoimmune diseases [39–41]. These results suggested a developmental period called a “window of opportunity”—when the microbiome that colonizes the newborn’s body from its local environment forms an immune system repertoire with lifelong consequences for the organism [42].
Such interaction in the early stages of postnatal development leads to the formation of an immune system adapted to the microbiota of the area in which the organism will grow in the future. And this development ends by puberty, after which the “early aging” of the immune system begins, about which so much has been written [43].
Post-pubertal age-related changes of the immune system
Such a close attention of gerontologists to the aging of the immune system was caused by the fact that age-related changes in the innate and adaptive immune system begin to develop earlier than other organs and systems, almost immediately after the onset of puberty [44, 45], although these changes may not be due to “aging” (in the sense of age-related changes), but to a feature of the postnatal development of the immune system, which is closely related to the aggressiveness of the external environment, the constant persistence of pathogenic viruses and bacteria, and the formation of a balance between the innate and adaptive immune systems [46]. In addition, their interaction with other organs and systems determines the development of the organism as a whole [47]. Interest in age-related changes in the immune system began with a conflict between the results of cell biologists and immunologists which demonstrated that T-cell proliferation in vitro can proceed indefinitely [48, 49] and is not limited by the Hayflick limit [50]. Later on, subsequent studies have shown that the average number of cumulative doublings of the T cell population decreases between 25 and 40 passages [51, 52] and its undergo replicative senescence in vitro as other cell cultures [53]. These and many other studies formed the basis of the research in the field of immunology of aging. Almost all of the possible mechanisms of age changes in the immune system have been found during this time. It was shown that stem cell functions are impaired with age. Hematopoietic stem cells lose their self-renewing and regenerative potential gradually with aging, while the frequency of cellular disorders in lymphocytes increases greatly. It was demonstrated that in aged mice, the pool of HSCs in the bone marrow has expanded significantly, but their differentiation potential is restricted [54, 55]. Age-related changes in the functions of the thymus are more pronounced. Thymus involution starts immediately after puberty and occurs in almost all vertebrates [56, 57]. Violation of the structure and functions of the thymus leads to a decrease in the production of naive T cells, which entails a disruption of the pool of peripheral T-cells, impaired activation of T cells in peripheral organs, and decreased immune response in general, and serves as a trigger for the decline in immune function with age [6, 58]. Such pronounced age-related changes in these two parts of the immune system became the first trigger for finding ways to rejuvenate the immune system.
Hematopoietic stem cells, lymphoid organs, and plasma transplantation
Various models of heterochronic chimeras became one of the first areas of research aimed at rejuvenating multiple organs, including the immune system: transplantation of hematopoietic stem cells, the microenvironment/niches of lymphoid organs, lymphocytes, blood plasma, which contains a number of systemic factors, was used for this [59].
The greatest interest among researchers was initially directed to bone marrow transplantation since age-related changes in their functionality were undeniably proven. The model of lethally irradiated heterochronic chimeras has been most widely used. Bone marrow cells were transplanted into animals of the opposite age after lethal irradiation, which ensured the preferential replacement of blood cells in the recipient with donor ones. The level of chimerism with donor green fluorescein protein positive (GFP+) cells reached 80% in the blood and bone marrow, 70% in the thymus, and 55% in the spleen of recipients 70 days after transplantation [60]. It was shown that the level of immune response to sheep red blood erythrocytes (SRBC) does not differ between groups of young lethally irradiated mice with transplanted young or old bone marrow cells. Conversely, the transfer of bone marrow cells from a young donor into the body of an irradiated old recipient did not restore the immune response of the old animal—it remained at the level of the old control animal [61]. Similar results were obtained in the study of subpopulations of lymphocytes in lethally irradiated heterochronic chimeras. It was found that young animals transplanted with old bone marrow cells had a reduced number of CD4+ and CD8+ T cells in the spleen compared to the group populated with young BM cells [62]. These data are confirmed by many other studies that reveal the impairment of HSCs functioning with age [63, 64]. On the other hand, changes in the functional properties of old HSCs did not significantly affect the amplitude of the humoral immune response to SRBC in young animals. This fact may indicate that age-related changes in HSCs cannot be the only cause of age-related disorders of the immune system. This assumption is also supported by the results showing that old HSCs lead to a more pronounced restoration of thymic mass and a proliferative splenocyte response to phytohemagglutinin (PHA) in old animals repopulated with old BM cells compared to young BM cells repopulation [62].
Attempts to prolong the life of mice by repeatedly transplanting bone marrow cells have also failed. It has been shown that three consecutive transplantations in total 125.1 ± 15.6 million BM cells from young donors to middle/old age recipients (beginning from 14-month-old) lead to the formation of 18.7 ± 9.6% of donor cell chimerism in the bone marrow of recipients. However, this young cell chimerism did not lead to a significant improvement in a number of parameters of the functioning of the immune system and did not improve the parameters of frailty, and pathomorphological results. In addition, lifespan did not differ significantly between the young bone marrow transplanted group compared to control mice [65]. These facts may indicate that the characteristics of the microenvironment of lymphoid organs, in which hematopoietic stem cells differentiate into mature immunocompetent cells, are key to the formation of immunological competence subsequently.
Thus, it was concluded that the only use of young bone marrow cells transplantation to rejuvenate the immune system is inefficient, probably due to the existence of an old body “factors” that block the complete differentiation and maturation of stem cells. One of these factors can be the niches of the lymphoid organs, in which the differentiation of lymphocytes occurs, as well as their interaction during the development of the immune response. Transplantation of individual lymphoid organs (thymus, spleen, and bone), or their combination with bone marrow transplantation, has also been used as a model for rejuvenating the immune system. It has been shown that transplantation of neonatal spleen, thymus, or their combinations in old animals does not improve the immunological parameters of the old immune system [66, 67]. Transplantation of young bone marrow simultaneously with transplantation of newborn thymus into old mice effectively restored impaired immune functions but did not affect the lifespan of mice. Sequential multiple transplantations of neonatal thymus, starting from adulthood, also effectively enhanced immunological functions, and reduced the incidence of tumors until the middle age of mice, but these effects leveled out when the mice reached the age of more than 24 months. It has been suggested that the neonatal thymus transplanted into the abdominal cavity underwent atrophy, which subsequently had a suppressive effect on the host’s immune system [68]. Although there is another possible explanation—this is the suppressive effect of the old thymus, which was revealed during its transplantation into newborn animals. It was shown that transplantation of 22-month-old thymus into thymectomized neonatal mice led to a significant inhibition of the amount of plaque-forming cells (PFCs) in response to SRBC immunization at 1, 2, 3, and 4 months after transplantation when compared with only thymectomy animals [69]. The mechanism of this suppression remains unexplored.
Another additional factor that can lead to an age-related decrease in immunological functions is the composition of the blood, which also changes significantly with age. The level and ratio of different hormones and other multiple humoral regulatory factors in the organism also serve as factors influencing the pathways of stem cell differentiation, lymphocyte functioning, and immune response formation [70, 71]. And it can have a negative impact on the level of proliferation of young hepatocytes, and hippocampal neurogenesis in young mice—these indicators decreased after transfusion of old blood [72]. However, the possibility of a rejuvenating effect occurring on the immune system resulting from the transfusion of young plasma into old animals is poorly understood. It was shown that chronic administration of young blood plasma twice a week to middle-aged mice for a year did not show a significant effect on the age-related decrease in the ratio of CD4/CD8 T-cells in the blood of CBA/Ca mice, and the incidence of age-related pathology of internal organs [73]. It was also shown that the parameters of some behavioral tests were improved [74]. The latter fact may indicate a negative effect of old plasma factors on the activity of immunocompetent cells, and the possibility of its correction by the Neutral Blood Exchange method, which is now actively developing.
Systemic blood and cells exchange in heterochronic parabiosis
As already mentioned above, a rejuvenating effect on individual fragments of the immune system—transplantation of HSCs, niches of lymphoid organs, and blood plasma, has a certain positive effect on individual immunological parameters but does not allow achieving a stable rejuvenation of the immune system to the level of a young animal in any of the experimental approaches. Therefore, a promising approach was to use the model of heterochronic parabiosis, which is a complex set of cellular and humoral factors that can have a targeted rejuvenating effect on the individual parts of the immune system. It should be noted that the model of parabiosis is a “biological symbiosis” of two animals that have a common capillary blood circulation created by surgery [75]. Despite limiting blood exchange between partners to only a massive area of capillaries, studies of blood flow kinetics and cell distribution among parabiotic partners found no evidence of a difference in blood leukocyte migration based on cell size or surface markers. In the study by Gibney et al. [76], all peripheral blood cell populations reached equilibrium within 14 days, and the average blood exchange rate between parabiosis partners was 16 μL/hour or 0.66% per hour. Other studies have also shown the presence of mutual migration of bone marrow stem cells [77], precursors of fibroblast, monocytes and macrophages in the lungs [78], and endothelial precursors [79] between partners. However, there are exceptions. In particular, the absence of migration of dermal fibroblast/myofibroblast progenitors between parabiosis partners was found [80]. The absence of cells of donor origin in such tissues as the epithelium of the skin and intestinal tract, muscles, brain, bones, and cells of the niches of lymphoid organs may indicate an isolated process of remodeling of these tissues due to local tissue-specific stem cells [81].
The study of the rejuvenation of the immune system on the model of heterochronic parabiosis showed that the migration of young hematopoietic stem cells in combination with young plasma factors did not contribute to thymus rejuvenation in old animals, it was not observed the recovery of the peripheral T cells repertoire, but only the regulatory T cells normalization. Moreover, all of these processes did not lead to immunological function restoration in the old parabiotic partners. On the contrary, it was observed a progressive decline in most immunological parameters in the young heterochronic partners in this model: the decrease of the thymus mass, age-related change of the peripheral T cells repertoire, and decrease of PHA-stimulated splenocyte proliferation [82, 83]. The most pronounced changes were found in the T-cell repertoire in young heterochronic partners. These changes were expressed by a decrease in the CD4/CD8 ratio, and an increase in the number of CD8+44+ and CD4+44+ memory cells in the spleen. The study of the mechanism of such rapid age-related changes in the immune system of young heterochronous partners showed that it may be associated with thymus atrophy [83], or disturbance in exposure to “aged” antigen-presenting cells of the microenvironment of lymphoid organs [84–86], or a paradoxical decrease in regulatory FoxP3+CD4+25+ T cells in old partners [83]. It is known that a decrease in the number of Tregs can lead to homeostatic T cell proliferation [87, 88]. Therefore, changes in the T cell repertoire of heterochronic parabionts can be mediated by impaired differentiation of Tregs, which in turn can provoke an increase in homeostatic differentiation of T cells and an increase in the number of T cells with a memory phenotype, suggesting compromising immunological functions subsequently [7].
Similar results were obtained in the study by Davies et al. [89], which also showed similar changes in immune parameters in peripheral lymph nodes observed in young heterochronic partners, practically without affecting the old one. Separation of parabiotic partners 4–5 weeks after pairing showed that old CD8+ T cells continued to persist in the blood of young recipients throughout the 3 months of the study due to a mechanism not yet understood (possibly homeostatic proliferation), which is supported by “old” lymphoid niches, and which plays an underestimated role in the aging of the immune system [89].
To understand how the identified disorders of immunological functions are irreversible and can be directly related to the aging of the immune system, it was decided to check how the coexistence in heterochronic parabiosis can influence life expectancy. So, single young and old mice were connected in parabiotic pairs for three months. Thereafter they were disconnected and their life expectancy was assessed. It was found that the number of young heterochronic mice began to decline after 8 months after disconnection, at the age of 14 months. Later their life expectancy continued to decline, reaching a significant difference with the control young isochronic group. The life expectancy of old heterochronic partners did not show any statistical differences with isochronic ones [90]. Therefore, it was suggested that the age-related changes in the immune system of young heterochronic partners might be part of the changes that lead to the real lifespan decrease and may be mediated by the systemic effects of the old organism.
In summary, single, intermittent, or chronic transplantation of lymphoid/hematopoietic cells, tissues, or biological fluids from young to old animals does not contribute to the sustainable rejuvenation of the immune system. It should be noted that the functioning of the immune system involves the proliferation and differentiation of lymphoid cells in situ in peripheral organs, and their interaction with the cells of the “old” niches, which, as a rule, determines the direction of their development. Obviously, cell therapy requires a more comprehensive approach that provides not only the rejuvenation of the pool of migrating lymphoid cells, but also the restoration of the functions of stromal cells that form the niches of lymphoid organs.
Systems-level impact on rejuvenating of the immune system
As mentioned above, the immune system has a multilevel structure, and most of the interactions between individual organs, lymphocytes, and body tissues are well understood within this system. At the same time, according to the data of the Biologydictionary.net [91], our body consists of at least 200 different types of cells, while cells of each type can be at different stages of proliferation/differentiation in the tissue at one time period. The development of such a complex self-organizing system as our organism leads to the close interaction of various organs and tissues, which allows them to synchronize many physiological processes, including the functioning of the immune system (Figure 1). The analysis of such impacts is extremely complex due to the need to study the interactions both within and between various physiological systems. At the same time, it is necessary to take into account age-related changes additionally, as well as the influence of environmental factors, such as nutrition, infectious diseases, vaccinations, physical activity, puberty, menopause, etc., which leave an imprint on each individual organism [92]. All of the above fits into the formulation of Walter Cannon’s concept of “homeostasis” (regulation of physiological processes with feedback and feed-forward). Homeostasis is actually a self-regulating process by which the body can maintain the stability of the internal environment, adapting both to changing external conditions, and age-related changes in various organs and systems. Understanding normal and pathological physiology, as well as aging, is impossible without understanding the concept of homeostasis, the violation of which leads to the development of pathology [93–95]. Analysis of experimental studies in cell cultures in vitro, and studies conducted on worms, flies, rodents, monkeys, and humans suggest a decrease in adaptive homeostasis with age, which is a potential factor in increased risk of aging-related diseases and mortality [96].
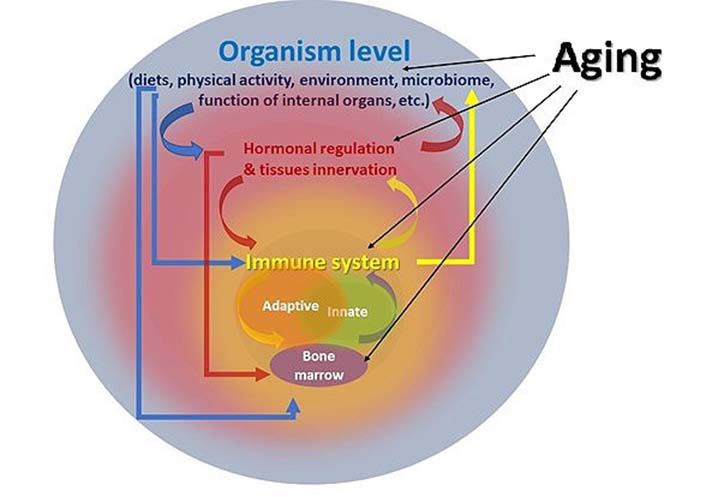
Multilevel interaction of the immune system with other functions of the organism during aging
One example of such a disruption of homeostasis is the chronic inflammation phenotype that appears with age, which formed the basis of the concept of immune aging [14]. Age-related immune inflammation is known to underlie the development of many pathologies—pathogenesis of osteoarthritis [97], cardiovascular diseases [98], atherosclerosis [99], diabetes [100], Alzheimer’s disease [101], age-related macular degeneration [102], and others. Many reviews have been devoted to the mechanisms of inflammation, in which the role of inflammation in the development of each individual pathology is analyzed in detail [15, 103]. The inflammation process starts after activation of pattern recognition receptors (PRRs) and consists of two main stages: detection of an exogenous pathogen, or any other endogenous structure (tumor cells, tissue damage, apoptotic cells, atherosclerotic plaques, etc.) [104]. After the activated cells secrete specific pro-inflammatory cytokines, most of which are aimed at enhancing the migration and differentiation of immunocompetent cells into the affected area [105]. Such a simple and understandable scheme for the development of the inflammation process is complicated by the fact that these receptors are expressed not only on innate immunity cells, but also on various other immune and structural cells of the body. They include endothelial and epithelial cells of the skin, intestines, and lungs; muscle and stromal cells, fibroblasts of various organs; as well as adipocytes and cells of the nervous system, and are expressed in almost all tissues—in the liver [106], blood vessel [107], skin [108], lungs [109], brain [110], and others. Therefore, the processes of inflammation can affect many vital functions of the body. Another complex aspect of inflammation is the incredible variety of cytokines produced during cell activation, which can also be produced by different types of innate and adaptive immune cells, as well as other cells of the body [111]. Cytokines produced during inflammation can be divided into two large groups—pro-inflammatory cytokines (which directly induce inflammation and tissue degeneration) and anti-inflammatory cytokines, the main function of which is to promote regeneration and healing processes. In general, the main goal of these two large groups of cytokines is not only to protect the internal environment of the body from exogenous influences but also to maintain the balance of tissue remodeling processes that constantly occur in the body throughout life [112–114]. With age, this balance is disturbed towards an increase in inflammation processes, however, the mechanism of its manifestation remains unresolved due to the complexity and variability of the changes taking place. In particular, one of these mechanisms is senescence-associated secretory phenotype (SASP)—a mechanism that suggests functional and/or phenotypic age-related damage in the cell structure of various tissues makes them targets for the immune system. In turn, different populations of leukocytes are involved in monitoring damaged (including through aging) cells, which leads to the emergence of SASP [115]. In fact, only the phenomenon that inflamm-aging appears with age has been recorded so far, but the trigger for its development remains a secret, closed in a black box. If return to the results described above, obtained using the model of heterochronic parabiosis, it could be assumed that the processes of inflamm-aging, which were launched in the old organism, cannot be prevented (or reversed) due to systemic factors, cells, and lymphoid niches of the young partner. And this process, which develops during aging, is so aggressive that more radical methods of combating it are required.
The lack of a clear understanding of the development mechanism of inflaming seriously complicates the development of approaches to combat this process. And, the only therapeutic approaches (which are currently offered to prevent inflammation) are aimed at blocking the production of inflammatory cytokines. In fact, this symptomatic treatment can eliminate the consequences for a short time but does not prevent the cause of inflammation—antagonists, and cytokine-neutralizing antibodies, anti-inflammatory drugs, and immune epigenetic targeting [116–118].
Paradoxically, a simpler and cheaper method of rejuvenating the immune system may be such a well-known approach as caloric restriction (CR), which includes reducing calorie intake or fasting. Indeed, CR extended lifespan by 50% of most model organisms tested, reduced aging-associated diseases, and preserved loss of brain white matter [119, 120]. CR also has a rejuvenating effect on murine NK cells and T lymphocytes [121], as well as the use of metformin, a CR mimetic, a hypoglycemic drug that gained its popularity after Anisimov’s publication on increasing life expectancy and on the development of spontaneous mammary tumors in HER-2/neu transgenic mice [122]. Later it was shown that Metformin treatment has a positive effect on the Th17 inflamm-aging profile of CD4 T cells in elderly humans by increasing autophagy and improving mitochondrial function actually switching the “old” pro-inflammatory T cells to a “young” phenotype [123].
It should be noted that the mechanism of life extension when using both of the above approaches is mediated by changes in sensitivity to growth hormone, insulin-like growth factor-1, and fibroblast growth factor 21 [124, 125]. And the most effective genetic intervention that increased the lifespan of laboratory animals is the knockout of growth hormone and/or insulin-like growth factor-1 genes [126]. Deletion of insulin receptor substrate 1 (Irs1) gene extends up to 32% median life span in female mice relative to wild type of mice (C57BL/6 background) and shows numerous delayed age-related parameters, including motor coordination, glucose and insulin resistance, total fat mass, and some immune values—naive and memory T-cell number in blood [127]. At the same time, a similar effect does not lead to positive changes in males, which may indicate a strong influence of sex hormones on ontogeny and aging of many body functions.
Similar results have been described in the study of immune functions in genetically modified Dwarf mice [128]. This Ames dwarf mutation (recessive mutation in the Prop 1 gene (Prop1df) in homozygous form is characterized by lack of growth hormone, prolactin, and thyroid-stimulating hormone, and extend longevity by 50% in both males and females [129]. In addition to life extension, Dwarf mice have been shown to significantly slow down the rate of immune system aging compared to their wild-type controls [128].
Another approach to increasing lifespan is the use of senolytics, substances that specifically eliminate senescent cells from the body and help reduce the inflammatory phenotype [130, 131]. And recently, the number of studies on the use of senolytic compounds to restore the immunological functions of the old organism has been progressively increasing. In particular, it has been shown that in old mice infected with a virus related to SARS-CoV-2, treatment with the experimental senolytic compound AP20187 reduces mortality and increases the amount of antiviral antibodies in the blood of animals [132]. Treatment with navitoclax or its combination with dasatinib or quercetin (senolytic-like drugs) alleviated COVID-19-like lung disease and reduced inflammation in hamsters and mice [133]. Treatment of mice with muscle atrophy with fisetin resulted in a decrease in the number of senescent immune cells and restoration of the muscle phenotype [134]. The justification for the use of senolytics as a treatment strategy for many age-related pathologies led to their practical application and the start of clinical trials [135].
Conclusions
Aging of the immune system is accompanied by a complex of disorders, which is primarily represented by atrophy of the thymus, switching the process of T-cell differentiation from the thymic to the non-thymic pathway, where T-cell differentiation occurs in peripheral tissues (skin and intestines). As a result, the specificity of the T-cell receptor decreases with age, which leads to a decrease in the specificity of the immune response in general and an increase in the number of autoimmune clones. In addition, another mechanism of aging is the phenotype of chronic inflammation, which formed the basis of the theory of inflammatory aging. The development of inflammatory aging is largely mediated by age-related changes in both lymphoid, antigen-presenting, and non-immune cells, such as fibroblasts and epithelial cells. All these cell types are localized in almost all tissues of the body, capable of producing a wide range of cytokines in response to tissue repair after damage or activation by antigen or PRR receptors, and regulating migration and antigen-specific differentiation of lymphocytes. This fact makes cell-mediated therapy ineffective, since the differentiation of hematopoietic stem cells, or naive lymphocytes in the old organism, occurs in the pro-inflammatory microenvironment of old organs. Local restoration of niches of lymphoid organs also does not give the desired effect. Although such approaches are actively discussed, in particular in the review by Borgoni S. et al. [118], the single elimination of individual dysfunctions of T- or B-lymphocytes, or NK cells, macrophages, dendritic cells or regeneration of the thymus may be ineffective for restoring immune functions in general in the elderly.
Can these difficulties be overcome? The use of therapeutic drugs aimed at prolonging life in general or overcoming the development of chronic inflammation in particular leads to a complex rejuvenation of the whole organism, including the immune system. Perhaps the use of senolytics that can affect both of these processes will become one of the promising areas in the near future.
Abbreviations
BM: |
bone marrow |
CR: |
caloric restriction |
HSCs: |
hematopoietic stem cells |
NK: |
natural killer |
SRBC: |
sheep red blood cells |
TCR: |
T-cell receptor |
Declarations
Acknowledgments
I thank Tatiana Dubilei and Olexyi Hryhorov for the creative discussions and comments on the manuscript.
Author contributions
IP: Writing—original draft, Writing—review & editing.
Conflicts of interest
The author declares that there are no conflicts of interest.
Ethical approval
Not applicable.
Consent to participate
Not applicable.
Consent to publication
Not applicable.
Availability of data and materials
Not applicable.
Funding
Not applicable.
Copyright
© The Author(s) 2023.