Abstract
Globally, the incidence of Parkinson’s disease (PD) is increasing faster than other neurodegenerative disorders. Neuropathologically, PD is characterized by the loss of dopaminergic neurons in the substantia nigra pars compacta due to the accumulation of aggregates of misfolded α-synuclein (α-Syn) in the cytoplasm of these neurons, forming Lewy bodies. Extracellular vesicles (EVs) are associated with the spread of α-Syn to different brain areas. However, at the same time that these EVs contribute to the pathophysiology of PD, they can also be explored as therapeutic, serving as a vehicle to deliver specific molecules, since these vesicles can easily cross the blood-brain barrier. Thus, this review summarizes the recent progress in EVs as a therapeutic strategy for PD, focusing on their delivery to the brain, and discusses the potential challenges and future directions in this field.
Keywords
Parkinson’s disease, extracellular vesicles, exosome, drug deliveryIntroduction
Parkinson’s disease (PD) is the second most common neurodegenerative disorder worldwide [1–3]. Epidemiologically, PD affects 1–2 people per 1,000 at any time. However, the prevalence of the disease increases with age, affecting about 1% of the population over the age of 65 years old [4], and 4% to 5% of the population over 85 years old [4, 5].
Neuropathological hallmarks of PD are loss of dopaminergic neurons in the substantia nigra (SN) pars compacta (SNpc), causing a dopamine (DA) deficiency at the striatum, and accumulation of aggregates of misfolded α-synuclein (α-Syn) into the cytoplasm of dopaminergic neurons, forming the structures known as Lewy bodies (LBs) [1, 2, 6].
Although the mechanisms leading to progressive neurodegeneration in PD remain not completely understood, they are believed to be intimately dependent on a complex and refined communication system that leads to gradual neuronal death [7, 8]. In addition to axonal and synaptic interactions, there is growing evidence of the crucial contribution of extracellular vesicles (EVs) to both the physiological functioning of the central nervous system (CNS) and the pathogenesis of neurodegenerative diseases [8].
EVs carry a plethora of biomolecules that have been associated with the activation of microglia and neurons and the promotion of the spread of α-Syn in PD, thus contributing to neuronal death [9, 10]. However, by carrying other proteins, metabolites, coding and non-coding RNAs, and organelles, such as mitochondria, these vesicles can be explored for PD treatment in an innovative and disruptive therapeutic approach known as cell-free therapy, as previously revised by Araldi et al. [9]. Furthermore, owing to their nanosized diameter, some types of EVs can easily cross the blood-brain barrier (BBB), serving as vehicles for both naturally produced biomolecules and synthetic drugs [11–15].
Based on these data, this review aimed to summarize the most recent advances in the biology of these EVs, describing the role of these vesicles in PD progression and exploring these nanosized vesicles as a novel class of therapeutics for PD treatment (cell-free therapy).
PD: from pathophysiology to the economic burden
Since aging remains the largest risk factor for developing PD, the economic and social impact of PD will continue to rise with the overall longevity of many populations [16]. According to a projection for 2037 in the United States, the total economic burden of PD is estimated to exceed $79 billion, emphasizing the need for novel therapies that can modify the natural history of the disease [17]. This is because, to date, no therapy can postpone or avoid neurodegeneration.
The prevalence of PD has more than doubled over the last two decades, making it the fastest-growing neurological disease [18, 19]. According to the Global Burden of Disease (GBD) study, 6.1 million PD patients were reported in 2016 globally, indicating an increase of 21.7% in the disease prevalence from 1990 [19]. Considering this rapid increase in the number of novel cases of PD registered annually, it is estimated that the number of cases will exceed 12 million by 2040 [19, 20].
PD is characterized by the loss of dopaminergic neurons in the SNpc in the midbrain with concomitant loss of axons that project to the striatum along the nigrostriatal pathway [21]. This degeneration results in the loss of the neurotransmitter DA, leading to the primary motor symptoms of PD (described for the first time by James Parkinson in 1817), which include bradykinesia, ataxia, tremor, rigidity, and postural instability [1, 3, 6]. Histologically, PD is characterized by the inclusions of misfolded α-Syn in neuronal cell bodies (known as LBs) and within neuronal cell processes (Lewy neurites) [1, 3, 4, 6].
In the last two decades, monogenic mutations in at least 20 genes have been linked to autosomal dominant or recessive forms of familial PD, accounting for less than 5% of all cases of the disease. Therefore, the remaining 95% of PD cases remain idiopathic [16], and aging represents the main risk factor for this type of PD [22].
Aging promotes biochemical and molecular changes in nigral dopaminergic neurons, leading to mitochondrial dysfunction, iron and calcium dysregulation, and antioxidant deficiencies [18, 23, 24]. Interestingly, mitochondrial dysfunction has been implicated in the pathogenesis of both monogenic and idiopathic PD [18].
In familial cases (monogenic), mutations in specific genes, such as SNCA (encoding α-Syn), PINK1 (encoding PINK1), and PRKN (encoding parkin) were also extensively related to mitochondrial dysfunction [18]. However, the N-terminal domain of α-Syn gives the protein a high affinity for the mitochondrial membrane [25]. This feature could explain why α-Syn is found in all mitochondrial compartments, regulating processes, such as mitophagy and mitochondrial fission and fusion [25].
In contrast to monomers, α-Syn oligomers are deleterious for ATP synthesis. The accumulation of these oligomers reduces complex I activity and increases reactive oxygen species (ROS) production, leading to oxidative stress [18]. This action not only reduces the energy supply but also activates microglia, leading to the production of inflammatory cytokines, such as tumor necrosis factor-α (TNF-α), interleukin (IL)-β, and IL-6 [26]. Neuroinflammation promotes neuronal damage and contributes to neuronal loss in PD [27, 28].
Although the first symptoms (motor symptoms) of the disease occur due to α-Syn accumulation in the basal ganglia (SNpc), PD begins in the lower brainstem and olfactory system (stage 1). Next, the disease moves further up the brainstem, traveling to the areas below the SNpc, resulting in non-motor symptoms, such as pain and mood (stage 2). Until the beginning of stage 3, occurs the formation of LBs within the SNpc. In stage 4, a large proportion of DA-producing cells are affected. However, the disease continues to spread to the limbic system, which is involved in emotions, motivation, and long-term memory. Next, the disease spreads to the mesocortex (the brain area between the limbic system and the cerebral cortex). By this stage (stage 5), the disease began to invade the neocortex (which is involved in higher-order brain functions such as perception, cognition, and language), affecting the memory and sensory areas in the brain [29].
EVs on PD
Although PD had been discovered more than two centuries ago, the physiopathology of the disease remained unclear for many years [30]. Nevertheless, great progress in the last decades has been made in understanding the genetic causes and molecular pathways of PD, which are helping to unravel important features of Parkinson’s pathogenesis [1, 2, 31].
The discovery of PD hallmarks, such as loss of dopaminergic neurons in the SNpc, accumulation of misfolded α-Syn, and formation of LBs, enabled the treatment of the motor symptoms of the disease through the replacement of DA, mainly by oral levodopa administration [1, 3, 6]. Despite the life quality improvement, the replacement of DA cannot stop the neurodegenerative process. In addition, the progression of the disease causes eventual resistance or lack of efficacy of conventional drugs, demanding other clinical and non-curative approaches [1, 17].
The development of novel therapeutic approaches depends on a better understanding of how different aspects of the pathology affect the development and progression of PD. In addition, given its multiple clinical presentations and grades of severity and behavior, it is argued that PD should have personalized and multidisciplinary therapies, depending on the degree of neurodegeneration in patients [6, 32]. Although the pathogenesis of PD is undoubtedly multifactorial, the most commonly investigated features of PD progression are the accumulation of α-Syn, as well as the increase of ROS production and mitochondrial dysfunction [3, 23].
In particular, mitochondrial dysfunction verified in PD has been established for over three decades and proposed as an early mechanism of pathogenesis [33]. After many years of research, this vital organelle still shows new evidence of its role in PD development [3, 31] and implications for the development of novel therapies [3, 34].
Important mitochondrial abnormalities related to PD include impairment of the mitochondrial electron transport chain, more specifically, complex I dysfunction, mitophagy defect, mitochondrial DNA (mtDNA) mutations, and changes in mitochondrial morphology [35, 36]. It is unclear whether the mitochondrial imbalance is the cause or consequence of PD. However, these known impairments lead to a dynamic bioenergetic imbalance, creating an environment of high metabolic stress sustained by a vicious cycle of mitochondrial damage [31]. Under regular conditions, mitochondrial stability and homeostasis are guaranteed by a quality control system called mitochondrial quality control (MQC), which allows the removal and replacement of damaged components [35]. However, MQC elements present different grades of dysfunction under certain conditions, such as aging [37, 38], PD-related genes (e.g., LRRK2, PINK2, PARK7/DJ-1) [39, 40], and ambient exposure to 1-methyl-4-phenyl-1,2,3,6-tetrahydropyridine (MPTP) and rotenone [3, 41].
In PD, mitochondrial dysfunction is believed to exacerbate the α-Syn in cascade, resulting in neuronal degeneration and LB formation [23, 31, 42]. Concurrently, α-Syn aggregation amplifies microglial activation, which attracts peripheral immune cells to the CNS. Subsequently, activated lymphocytes elicit prolonged and compromised microglial activation, which could potentially facilitate both mitochondrial dysfunction and neuronal degeneration [43–45]. Collectively, these events show that the onset and progression of PD are dependent on complex cellular and environmental networks [7, 8]. Given their crucial role in mediating cell communication and their intrinsic involvement in the physiological functions of the CNS, emerging evidence indicates that EVs may be closely linked to pathophysiological conditions and neurodegenerative diseases [7, 8]. EV is a generic term that englobes diverse subtypes of vesicular structures released by cells, including, but not limited to, exosomes, microvesicles, microparticles, ectosomes, oncosomes, apoptotic bodies, and numerous other nomenclatures [46]. In recent years, the classification of EVs has primarily been based on their origin (cell type), size, morphology, and cargo content [47]. In terms of size, EVs exhibit a size spectrum ranging from 50 nm to 1,000 nm in diameter, with the potential to surpass the upper limit of this range in the case of oncosomes, which can reach dimensions of up to 10 µm [47].
EVs can also be classified into two main groups based on their intracellular origin: 1) exosomes, which are formed within the endocytic pathway generated by invagination of the endosomal membrane, which creates multivesicular bodies (MVBs) capable of fusing with the plasma membrane to discharge exosomes into the extracellular milieu [48–50], and 2) microvesicles, formed by the outward budding and fission of the plasma membrane [48].
Exosomes are the most widely investigated subtype of EV, with a diameter ranging from 30/40–100/150 nm [47, 51, 52]. The ability of this subtype of EV to transport bioactive components and overcome biological barriers has led to considerable interest and an increasing number of investigations regarding exosome roles in pathophysiological processes, as a biomarker of disease, and as a cell-free therapeutic agent [50, 53, 54].
Concerning PD, many studies have shown that exosome content in the CNS changes during disease and can be found in cerebrospinal fluid and peripheral body fluids [51, 55, 56]. Different groups have shown differential exosomal content, such as microRNA (miRNA) and α-Syn, between circulating exosomes of healthy and PD individuals, pointing out exosomes as a promising candidate for biomarker development in PD [56–60]. Regarding PD’s physiopathology, exosomes (along with other EV subtypes) secreted by activated microglia and neurons have been implicated in the progression of PD by promoting the spread of α-Syn and increasing neuroinflammation, thus exacerbating neuronal death [9, 10].
Despite the special focus on exosomes in a great part of the literature, it is crucial to consider an important point for the continuation of this review: EVs present an overlapping range of sizes, similar morphologies, and variable compositions, depending on their cells of origin, features that challenge the precise nomenclature of isolated EVs [46, 47]. Isolation of exosomes is usually performed by differential centrifugation, which separates vesicles by size and/or density. Thus, the vesicle content is not likely to be completely homogeneous and is constituted by only one subtype of EV [46, 48, 61, 62].
In this sense, to overcome the problem of inaccurate denominations of EV subtypes, the International Society for Extracellular Vesicles (ISEV) endorses the usage of more general terms, considering the physical characteristics (e.g., size or density), biochemical composition (e.g., CD63 positive staining), or descriptions of cell origin or cell conditions (e.g., apoptotic bodies, hypoxic EVs) [46].
Given the previous information, the ISEV size-based nomenclature will be adopted in the current review to address the therapeutic possibilities of EVs in PD. According to the ISEV, EVs can be divided into “small EVs” (sEVs, ≤ 200 nm) and “medium/large EVs” (m/lEVs, > 200 nm), as illustrated in Figure 1 [46].
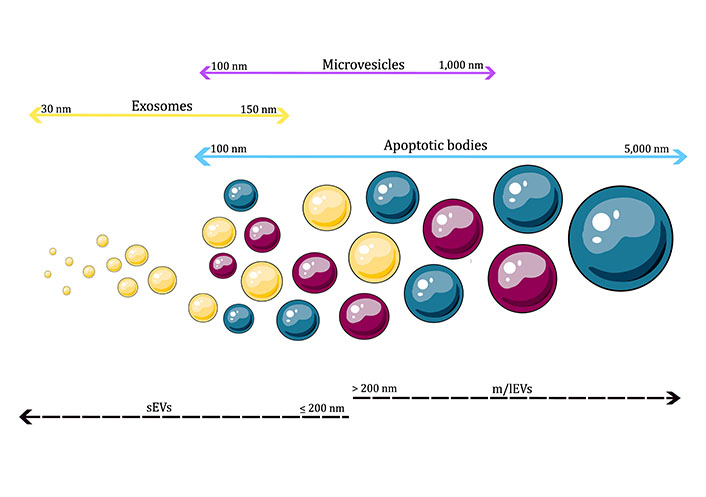
Classification of EVs by size. sEVs are vesicles ≤ 200 nm, while m/lEVs are vesicles > 200 nm in diameter. As shown in the images, different types of EVs can overlap in size, thus challenging the classification of EVs exclusively by size
Note. This figure contains (modified) images from Servier Medical Art (https://smart.servier.com) and BioIcons (https://bioicons.com). CC BY.
Progress in the therapeutic use of EVs for the treatment of PD
EVs play a crucial role in cell-to-cell communication at both local and systemic levels in healthy and diseased conditions [63–65]. In the therapeutic context, EVs provide a promising way to deliver proteins, messenger RNAs (mRNAs), miRNAs, or drugs to the CNS owing to their inherent ability to transport molecules between cells, unique immunological features, stability of their contents, and capability to cross the BBB, making them a potential therapeutic tool for neurodegenerative diseases, such as PD [12, 14, 15].
In the following sections, the main advances regarding the two approaches to the use of EVs in PD will be discussed in more detail.
Therapeutic approaches based on unmodified EVs
Unaltered EVs exert therapeutic effects through their intrinsic biological properties [49]. In addition, almost all cell types secrete these vesicles, and the cell composition and function are directly related to the EV contents [11, 50, 66]. Therefore, it is desirable to use EVs derived from cells with known or potential therapeutic effects [67]. Among these, stem cells, especially mesenchymal stem cells (MSCs), have been the most explored and investigated cell type in recent decades as a therapeutic option for countless incurable pathologies, including neurodegenerative diseases [32, 67–69]. Some mechanisms by which stem cells can exert their therapeutic effects include differentiation into various cell types, which makes them a potential source of replacement cells for damaged or diseased tissue; production and release of a plethora of molecules and biological [68].
For this reason, MSCs are a widely explored cell type used as a source of EVs for therapeutic purposes in PD. Among the various types of MSCs, the most used are those derived from dental pulp, umbilical cord [70, 71], bone marrow (BM), and Wharton’s jelly [72].
EVs secreted by these cell types carry therapeutic RNAs and proteins, which can provide neuroprotection, reduce oxidative stress and neuroinflammation, increase neurogenesis, and improve cognitive and mood functions in disease states [55, 73]. The following section summarizes several in vivo and in vitro studies that have investigated the potential therapeutic effects of unmodified MSC-derived EVs from different sources in PD models.
Dental pulp of stem cells from human exfoliated deciduous teeth
Jarmalavičiūtė et al. [74], used in vitro experiments to verify whether EVs isolated from stem cells from human exfoliated deciduous teeth (SHEDs) had therapeutic potential for the treatment of PD. The immortalized human neural progenitor cell line was differentiated into a dopaminergic neuronal phenotype and exposed to the neurotoxin 6-hydroxydopamine (6-OHDA) to mimic the parkinsonian/oxidative environment. This study demonstrated that SHED exosomes, but not microvesicles, suppressed 6-OHDA-induced apoptosis in dopaminergic neurons, suggesting that SHEDs-derived exosomes may represent a promising novel therapeutic tool for the treatment of PD [74]. Subsequently, in 2019, Narbute et al. [75] investigated the therapeutic effects of intranasal administration of SHEDs-derived EVs in a rat model of PD induced by 6-OHDA medial forebrain bundle (MFB) lesions. The results showed that EVs effectively suppressed 6-OHDA-induced gait impairments and contralateral rotations in the apomorphine test and normalized tyrosine hydroxylase expression in the striatum and SN of rats.
Human umbilical cord MSCs
Chen et al. [71] demonstrated cytoprotection in an in vitro model of PD (SH-SY5Y cells treated with 6-OHDA) after co-incubation with human umbilical cord MSCs (hUC-MSC)-derived sEVs. The same study investigated the in vivo effect of these sEVs using a rat model of PD (lesion induced by 6-OHDA) and showed that hUC-MSC EVs can cross the BBB and reach the SN, leading to a reduction in dopaminergic neuron loss and apoptosis and increased DA levels in the striatum, decreasing the asymmetric rotation induced by apomorphine [71]. In a more recent study, EVs derived from hUC-MSCs and rich in miRNA-106b showed the potential to inhibit neuronal apoptosis and increase autophagy in a mouse PD model (lesion induced by intraperitoneal injection of MPTP) [70]. In an in vivo study, Zhang et al. [76] observed an improvement in behavior, a reduction in neuronal damage, and an increase in the concentration of striatal DA and its metabolites after treatment with hUC-MSC-derived EVs, effects hypothesized to be mediated by the inhibition of excessive microglial proliferation.
BM-MSCs
BM-MSC-derived EVs enriched with wingless/integrated 5 (Wnt5) have been shown to rescue the rotation behavior and climbing speed of rats in the 6-OHDA model, possibly by repairing damage to DA nerves and remodeling the inflammatory microenvironment in the SN [77]. Additionally, the same type of vesicle (BM-MSC-derived) positively affected the expression of circadian rhythm-associated genes and increased the levels of DA and 5-hydroxytryptamine, indicating their potential for treating sleep disorders associated with PD [78].
In summary, the described studies, and those listed in Table 1, reinforce the therapeutic features of MSC EVs as a promising tool for PD and other neurodegenerative disorders. Altogether, these EVs have shown the capability to protect and restore DA neurons, reduce neuroinflammation, and even improve symptoms related to sleep disorders associated with PD [70, 71, 74–77].
Studies utilizing unmodified EVs on PD models
Therapeutic cargo | EV source | Experiment type | Ref. |
---|---|---|---|
Natural EV-cargo | SHEDs | In vitro | [74] |
Natural EV-cargo | SHEDs | In vivo | [75] |
Natural EV-cargo | hUC-MSCs | In vitro and in vivo | [71] |
Natural EV-cargo but rich in miRNA-106b | hUC-MSCs | In vitro and in vivo | [70] |
Natural EV-cargo but rich in Wnt5 | BM-MSCs and BM-MSC-derived DA neuron | In vivo | [77] |
Natural EV-cargo | BM-MSCs | In vivo | [79] |
Natural EV-cargo but rich in Wnt5 | BM-MSCs and BM-MSC-derived DA neuron | In vivo | [78] |
Natural EV-cargo | hUC-MSCs | In vitro and in vivo | [76] |
Although these results are promising, clinical studies on PD have not yet been conducted in humans. This is likely because of safety and efficacy concerns, regulatory hurdles, ethical considerations, and financial challenges [80, 81]. For these reasons, further research is needed to fully understand the mechanisms underlying the therapeutic effects of MSC-derived EVs and, above all, clarify the safety of this type of intervention.
Therapeutic approaches based on engineered and nanocarried EVs
EVs have emerged as potential therapeutic agents for treating various diseases, including PD. They offer several advantages over synthetic drug carriers such as liposomes and nanoparticles. These advantages include facile loading with therapeutic content, the ability to achieve site-specific delivery, natural biocompatibility, and the inherent ability to transport molecules across biological barriers [48, 82–84].
EVs can be modified to enhance their therapeutic potential by either modifying EV-producing cells or by modifying isolated and purified EVs. The former approach includes strategies such as genetic engineering to increase the quantity of the desired cargo or express specific membrane markers, which can improve the targeting ability of EVs. The latter approach involves the loading of EVs with the desired cargo, which can be achieved using techniques such as co-incubation, electroporation, and sonication [85–87]. Furthermore, EVs can be modified by targeting ligands such as specific antibodies, peptides, and chemical structures to augment their delivery efficiency, biodistribution, and pharmacokinetic properties (Figure 2) [64, 88–90].
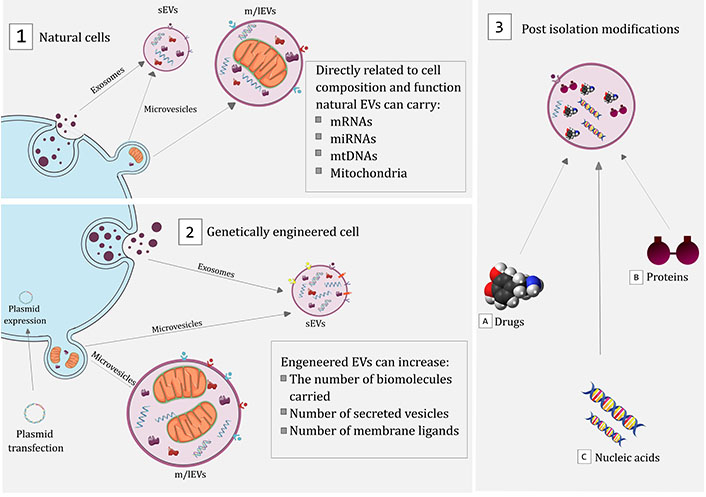
Therapeutic approaches for EV usage in PD treatment. Schematic representation showing the main strategies proposed to explore the therapeutic potential of EVs: (1) EVs, such as microvesicles and exosomes, can be secreted into the extracellular environment, carrying biomolecules directly related to the profile and function of the secreting cell; (2) cells can also be genetically modified using plasmids that can induce the production of biomolecules that are not naturally produced by transfected cells or increase the natural production of certain biomolecules; (3) after isolation, EVs can also be modified, allowing the introduction of drugs (e.g., DA), proteins (e.g., catalase), or nucleic acids (e.g., aptamers)
Note. This figure contains (modified) images from Servier Medical Art (https://smart.servier.com) and BioIcons (https://bioicons.com). CC BY.
Several studies have demonstrated the potential of loaded EVs to deliver therapeutic agents for PD treatment [87, 91, 92]. Haney et al. [87] utilized EVs as carriers for catalase delivery in PD. Catalase is an efficient antioxidant that can reduce ROS and prevent oxidative damage to cells, which plays a significant role in PD pathogenesis. However, the therapeutic applications of catalase are limited by its low bioavailability and short half-life in the body. In this study, EVs were isolated from the RAW 264.7 macrophage cell line, and five methods were tested for catalase loading: co-incubation, permeabilization with saponin, freeze-thaw cycles, sonication, and extrusion. This study showed that catalase-loaded EVs were efficiently taken up by neuronal cells in vitro and were detected in the brains of 6-OHDA mouse PD models after intranasal administration. Furthermore, it provided significant neuroprotective effects in both in vitro and in vivo PD models because of its high loading efficiency, sustained release, and preservation of catalase against protease degradation [87].
EVs have also demonstrated the potential to deliver drugs with poor pharmacokinetic properties. For example, in the context of PD, Peng et al. [91] demonstrated the use of EVs to deliver curcumin to the brain. Curcumin, a compound with a high antioxidant capacity, has potential therapeutic effects in several diseases, including PD [93]. However, the poor pharmacokinetic properties of curcumin have limited its therapeutic potential [92]. To overcome this, the authors developed a self-oriented nanocarrier called PR-EXO/PP@Cur, which combined curcumin with MSC-derived exosomes. This approach demonstrated the reduction of α-Syn aggregates, recovery of neuronal function, and alleviation of neuroinflammation in vitro and PD mouse models [91].
EVs are appealing for therapeutic purposes not only because they can be loaded with desired compounds, but also because of their natural ability to cross the BBB. Additionally, this ability can be further amplified through certain modifications [94, 95]. In this sense, EVs can be a powerful tool to improve drug treatment, as one of the most frequent problems with drugs to treat neurological problems is the poor ability of some of these drugs to cross the BBB [96]. To further increase the ability of EVs to cross the BBB, Qu et al. [97] developed a strategy that involves pre-treating EVs with transferrin to stimulate interaction with the transferrin receptor present on the BBB. In this study, EVs were isolated from the blood, loaded with DA via co-incubation, and administered intravenously to a 6-OHDA PD mouse model. This study demonstrated that pre-treatment of EVs with transferrin can enhance their ability to cross the BBB and that EVs can represent a viable means of delivering DA to the brain, a molecule of interest for PD treatment but with low BBB permeability [97].
To enhance the specificity of EV delivery to brain cells, one approach is to modify EV-producing cells through genetic engineering to express surface markers that promote binding to brain cells [88]. An example is the development of a molecular system involving the fusion of the peptide rabies viral glycoprotein (RVG) to the N-terminus of the exosomal protein lysosome-associated membrane glycoprotein 2b (Lamp2b). RVG specifically binds to the acetylcholine receptor in target cells, whereas Lamp2b is a protein found abundantly on exosomal membranes. This Lamp2b-RVG construct was used to transfect EV-producing cells in previous EV isolation [85]. In this regard, studies have implemented this system for the treatment of PD [98–101]. The first study, conducted by Cooper et al. [98], utilized dendritic cells transfected with the Lamp2b-RVG construct to isolate EVs. These EVs were loaded with α-Syn small interfering RNA (siRNA) via electroporation. The efficacy of this approach was assessed using in vitro and in vivo models. The results demonstrated a reduction in α-Syn mRNA levels and intraneuronal misfolded protein aggregation, highlighting the potential of this approach for therapeutic interventions targeting PD [98]. In another study, EVs were isolated from HEK293T cells transfected with Lamp2b-RVG plasmid [99]. The researchers then employed a co-incubation methodology to load the EVs with aptamers. Aptamers are single-stranded oligonucleotides that can bind to molecules and biological structures like antibodies [99]. In this study, EVs were loaded with aptamers capable of binding to α-Syn. After systemic administration of these EVs to α-Syn preformed fibril (PFF)-inoculated wild-type mice, there was a significant reduction in pathological α-Syn aggregates in the brain, and the associated motor dysfunction was rescued [100].
Kojima et al. [101] developed a set of EXOsomal transfer into cells (EXOtic) to enable efficient and tailored production of designer exosomes in engineered mammalian cells. These devices enhance exosome production, specific mRNA packaging, and the delivery of mRNA into the cytosol of target cells. The EXOtic approach was applied in PD using HEK293T cells to increase the expression and packaging of catalase mRNA in their EVs. EVs produced with this platform deliver therapeutic catalase mRNA to the brain and reduce neurotoxicity and neuroinflammation in vitro and in 6-OHDA mouse PD models, indicating the potential usefulness of EXOtic devices for RNA delivery-based therapeutic applications [101].
Overall, the works gathered here provide in vitro and in vivo evidence of the potential use of EVs as a tool in the treatment of PD (Table 2). EVs have proven to be an excellent platform for drug delivery, mainly because of their ability to transport molecules across biological barriers, site-specific delivery, and easy loading with therapeutic content [79, 98, 102].
Studies utilizing modified EVs on PD models
Therapeutic cargo | EV source (cell line or biofluid) | Modification | Loading method | Ref. |
---|---|---|---|---|
α-Syn siRNA | Dendritic cell | Lamp2b-RVG | Electroporation | [98] |
Catalase | RAW 264.7 | Unmodified | Co-incubation, saponin permeabilization, freeze-thaw cycles, sonication, and extrusion | [87] |
Catalase mRNA | HEK293T | Lamp2b-RVG | n/a | [101] |
DA | Blood | Transferrin pretreatment | Co-incubation | [97] |
Anti-α-Syn shRNA-MCs | Dendritic cell | Lamp2b-RVG | Electroporation | [102] |
Aptamer F5R1 and F5R2 | HEK293T | Lamp2b-RVG | Co-incubation | [100] |
Natural EV-cargo with curcumin | MSCs | SA-P and SA-RVG | Sonication | [91] |
n/a: not applicable; shRNA-MCs: short hairpin RNA minicircles
Exploring PD through bioenergetic maintenance: delivery of mitochondrial contents via EVs
PD has been linked to mitochondrial dysfunction and impairment of bioenergetic metabolism [103]. Evidence suggests that mitochondrial trafficking naturally occurs between CNS cells [104, 105]. The transfer of mitochondria facilitates the introduction of healthy mitochondria from an external source, replacing damaged mitochondria, and restoring proper mitochondrial function [104].
Recent studies have demonstrated that mitochondria are horizontally transferred between mammalian cells, promoting their incorporation into the endogenous mitochondrial network of recipient cells and inducing changes in the bioenergetic profile and functional properties of the recipient cells [105]. In addition, the horizontal transfer of mitochondrial genes can lead to important cellular events, such as the initiation of stem cell differentiation, reprogramming of differentiated cells, and activation of inflammatory signaling pathways. Among other cellular events, intercellular mitochondrial transfer can be mediated by tunneling nanotubes (TNTs), mitochondrial ejection, cytoplasmic fusion, and EVs [105].
An increasing number of studies have demonstrated the important effects of mitochondrial transfer in vivo and in vitro models of various disorders related to mitochondrial dysfunction [13, 104, 106–109]. Recently, Chang et al. [106] delivered mitochondria both in their unmodified state (Mito) and conjugated them with Pep-1 (P-Mito) in rats with 6-OHDA-induced lesions (a PD animal model). Intranasal delivery of these mitochondria improved the rotational and locomotor behaviors of the Parkinson’s animals compared to those of the control groups. Additionally, DA neuron survival was observed in lesions of the SN and striatum in Mito and P‑Mito rats, and was attributed to the restoration of mitochondrial function and a reduction in oxidative damage in the lesioned SN [106].
Under physiological conditions, entire mitochondria can be loaded onto EVs [104, 108]. EVs are an attractive delivery method for mitochondria and their contents in various cell types because of their ability to interact with recipient cell membranes, facilitated by the expression of surface proteins such as integrins and tetraspanins [13]. Numerous studies have demonstrated the potential of mitochondria-loaded or mtDNA-loaded EVs to enhance bioenergetics, reduce mtDNA damage and inflammation, and increase cellular ATP levels [104, 108, 109]. Among the various sources of mitochondrial EVs, MSCs have emerged as a promising option. Interestingly, it has been hypothesized that the therapeutic effects of stem cells, such as cell-triggered repair, may be mediated through mitochondrial transfer [87, 110].
As an alternative to using unaltered cells as sources of mitochondria-containing EVs, Dave et al. [107] demonstrated an alternative approach by activating peroxisome proliferator-activated receptor-γ coactivator-1α (PGC-1α), a mediator of mitochondrial biogenesis, to increase the number of mitochondria-loaded EVs released by various cell types. This discovery introduces a novel method for utilizing mitochondria-containing EVs as a therapeutic tool [107].
Despite evidence supporting the benefits of mitochondrial transfer in PD, such as the restoration of mitochondrial function in affected cells, halting disease progression, and improving brain function [106, 111], there is a lack of studies investigating the therapeutic potential of mitochondrial transfer mediated by EVs in PD. Therefore, exploring these two tools together could provide an effective and possibly targeted delivery of mitochondrial contents, which deserves more attention as a therapeutic option for PD.
EVs as a viable therapeutic option for PD and other diseases: overcoming challenges and improving technology
EVs have emerged as a promising therapeutic approach for various diseases and have demonstrated efficacy in more than 30 different conditions [112]. However, several challenges must be overcome for the successful clinical translation of EVs.
Technical challenges in the production, isolation, and characterization of EVs include scalability, production efficiency, standardization of isolation, quality control, and storage conditions [15, 113]. The limited characterization capability of the product can complicate and increase the risk of the translation process, similar to other nanoparticle-based products [114]. Culture conditions such as cell passage, cell density, and EV collection frequency can also influence product quality, including yield, EV composition, and bioactivity. Therefore, the entire production process must be thoroughly standardized [115].
Safety is a critical aspect of any therapeutic intervention and data on the safety of EV-based therapies are limited. Although EVs are generally considered safe because of their biocompatibility and low immunogenicity, studies using animal models frequently do not provide safety data related to the use of these interventions. Further studies are required to evaluate the safety and potential risks of EV-based therapies in preclinical models, particularly toxicity, and understand the absorption, distribution, metabolism, and excretion profiles of EVs [15, 113]. This may help to clarify the safety aspects for future clinical investigations.
Finally, the biological mechanisms by which EVs exert their therapeutic effects are poorly understood, posing a challenge to their clinical application [89]. EVs have diverse and complex cargoes, enabling them to provide therapeutic potential for addressing various complex pathological processes. However, owing to the inherent complexity of EVs, establishing their mechanisms of action is challenging [112].
Conclusions
In summary, both in vitro and in vivo studies have provided evidence that EV derived from various tissue sources (particularly MSCs) can be biotechnologically explored for PD treatment. This is because, even unmodified, these vesicles carry a plethora of bioactive molecules that can simultaneously target different biological pathways which are naturally found dysregulated in PD patients. In this sense, cumulative evidence has shown that EVs reduce oxidative stress and neuroinflammation, as well as improve motor, cognitive, mood, and sleep functions associated with PD [1–4]. Additionally, studies demonstrating the potential of modified EVs to deliver various substances offer new possibilities for drug delivery that can help slow neurodegeneration and/or treat PD symptoms [95, 97, 101]. Overall, these results demonstrate that EVs are innovative and valuable tools for understanding the pathophysiology of PD and developing new therapies. However, despite the benefits provided by in vitro and preclinical studies, clinical studies evaluating the safety and benefits of cell-free therapy are required to confirm the therapeutic potential of these EVs.
Abbreviations
6-OHDA: |
6-hydroxydopamine |
BBB: |
blood-brain barrier |
BM: |
bone marrow |
CNS: |
central nervous system |
DA: |
dopamine |
EVs: |
extracellular vesicles |
EXOtic: |
EXOsomal transfer into cells |
hUC-MSCs: |
human umbilical cord mesenchymal stem cells |
ISEV: |
International Society for Extracellular Vesicles |
Lamp2b: |
lysosome-associated membrane glycoprotein 2b |
LBs: |
Lewy bodies |
m/lEVs: |
medium/large extracellular vesicles |
miRNA: |
microRNA |
mRNAs: |
messenger RNAs |
MSCs: |
mesenchymal stem cells |
mtDNA: |
mitochondrial DNA |
PD: |
Parkinson’s disease |
ROS: |
reactive oxygen species |
RVG: |
rabies viral glycoprotein |
sEVs: |
small extracellular vesicles |
SHEDs: |
stem cells from human exfoliated deciduous teeth |
SN: |
substantia nigra |
SNpc: |
substantia nigra pars compacta |
Wnt5: |
wingless/integrated 5 |
α-Syn: |
α-synuclein |
Declarations
Acknowledgments
The authors acknowledge Servier Medical Art (https://smart.servier.com) and BioIcons (https://bioicons.com) for providing the icons used for the images. Icons were developed by Servier and DBCLS and licensed under Creative Commons Attribution 3.0 Unported License and Creative Commons Attribution 4.0 International License, respectively.
Author contributions
MRT and ALA equally contributed to: Conceptualization, Writing—original draft, Visualization. VRdC and JRDP: Writing—original draft. RPA: Supervision, Writing—review & editing.
Conflicts of interest
The authors declare that they have no conflicts of interest.
Ethical approval
Not applicable.
Consent to participate
Not applicable.
Consent to publication
Not applicable.
Availability of data and materials
Not applicable.
Funding
Not applicable.
Copyright
© The Author(s) 2023.