Abstract
Earth’s rotation generates the basic circadian rhythm of day and night to which all living organisms must adapt to survive. In mammals, this happens thanks to a central clock located in the suprachiasmatic nucleus (SCN) of the hypothalamus and to peripheral clock genes at the cellular level. The main environmental cue capable of synchronizing such clocks is light sensed by retinal ganglion cells signaling through a complex nervous pathway to the pineal gland which ultimately regulates melatonin synthesis that occurs during the night, darkness hours in all mammals. The central clock synchronized by melatonin drives the circadian oscillation of the sympathetic nervous system (SNS) adrenergic activity which in turn controls glucocorticoid production in the adrenal glands. These oscillations are integrated with peripheral cellular clocks by still not completely understood mechanisms and drive the homeostatic control of activity-rest (sleep) cycles, cardiovascular activity, body temperature, and immune-hematopoietic functions. The neuronal and hormonal mechanisms governing the circadian oscillation of hematopoiesis and immunity will be addressed in this review focusing on those offering therapeutic perspectives.
Keywords
Suprachiasmatic nucleus, melatonin, clock genes, circadian rhythm, hematopoiesis, immune responseIntroduction
Life has evolved on Earth under the influence of an orchestra of circadian, seasonal, lunar, and solar cycles or rhythms. This eventually resulted in their incorporation into many fundamental physiological mechanisms. This integration has been achieved by the development of a network of endogenous biological oscillators synchronized to environmental cues, such as variation in light intensity, activity-rest cycles, and nutrition, that are essential in the organization of the basic biological mechanisms and play a crucial role in health and disease [1]. At the cellular level, this is achieved by clock genes, circadian locomotor output cycles kaput (Clock), brain and muscle ARNT-like 1 (Bmal1), period (Per), and cryptochrome (Cry) which have to be entrained in order to generate the circadian rhythm that has a 24 h cycle. This circadian oscillation is based on negative feedback exerted by the protein resulting from an activated gene on the related messenger RNA (mRNA) transcription [2]. In mammals, the suprachiasmatic nucleus (SCN) of the hypothalamus is the master circadian clock that directs physiological and behavioral rhythms influencing also peripheral oscillators incorporated in the circadian machinery by nervous and hormonal signals [3, 4]. The rhythm of the SCN is generated by the cyclical expression of clock genes in both its neurons and astrocytes regulated by reciprocal signaling. In particular, neurons are active during the daytime while astrocytes downregulate neurons during the nighttime by controlling extracellular glutamate concentration [5].
Light is the most efficient environmental cue which synchronizes the expression of clock genes and the SCN activity in all mammals. The retinal ganglion cells expressing melanopsin are the initial circadian photoreceptors and photic information gets directly at the SCN via the retino-hypothalamic tract. Melanopsin is activated by blue light (460–480 nm wavelength) and the ensuing signal regulates melatonin synthesis in the pineal gland by entraining the SCN. The related nervous pathway involves the paraventricular nucleus (PVN) of the hypothalamus, the intermediolateral cell column, and the superior cervical ganglia (SCG). Melatonin produced in the pineal gland upon the adrenergic activation of beta- and alpha-adrenoceptors expressed on pinealocytes is immediately released into the third ventricle and by entering the blood circulation acts on both the SCN and peripheral tissues to modulate the circadian activity (Figure 1) [6–8]. Remarkably, melatonin concentration is highest during the darkness hours in both nocturnal and diurnal species.
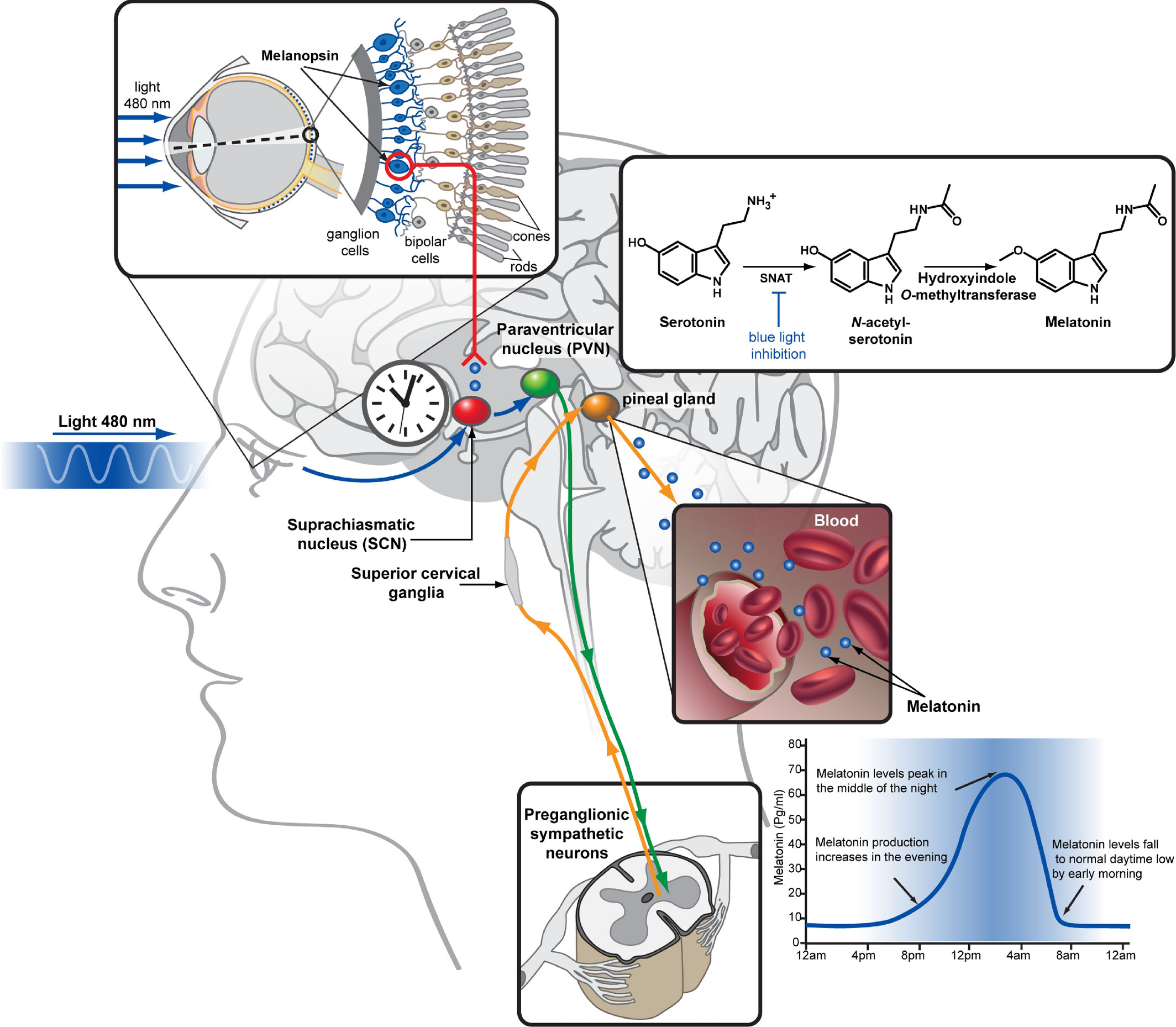
Melatonin biosynthesis. Serotonin is converted to melatonin in the pineal gland of the animal brain via two subsequent enzymatic reactions. The first step, N-acetylation, is catalyzed by serotonin N-acetyltransferase (SNAT) and inhibited by blue wavelength light signals transmitted from the retina via the SCN resulting in a high-level production of melatonin during the night and low level during the day. Melatonin is then released to the third ventricle and to the bloodstream, allowing it to act on both brain and peripheral tissues where melatonin type 1 receptor (MT1) and MT2 are expressed. Among the diverse physiological functions of melatonin is the regulation of body temperature and blood pressure, facilitating sleep
Note. Reprinted with permission from “Structural insights into melatonin receptors,” by Stauch B, Johansson LC, Cherezov V. FEBS J. 2020;287:1496–510 (https://febs.onlinelibrary.wiley.com/doi/full/10.1111/febs.15128). © 2019 Federation of European Biochemical Societies.
Probably the capability to synthesize melatonin (N-acetyl-5-methoxy-tryptamine) first appeared in bacteria living about 2.5 billion years ago in order to tolerate increasing concentrations of oxygen in the atmosphere. The bacteria were then internalized in eukaryotes probably by phagocytosis, and during evolution, they finally evolved in mitochondria [9]. This would explain why melatonin may be synthesized in many extra-pineal tissues and organs including lymphoid organs and the bone marrow (BM) [10, 11] where its production does not seem to follow any circadian rhythm. It also does not enter the blood circulation in a substantial amount. In these extra-pineal organs, melatonin works as an antioxidant or as an autocrine or paracrine substance. Melatonin is a multitasking molecule and as such it has both receptor-dependent and receptor-independent actions. The indoleamine which binds and activates MT1 and MT2, influences many physiological effects through G protein-coupled receptors. The extracellular signal of melatonin is transduced into intracellular signals affecting gene expression and protein posttranslational modifications. This action seems to be organ-specific and depends on the density of specific receptor expression [12]. It has also been suggested that melatonin may bind retinoic acid-related orphan receptors in the nucleus although this finding was questioned by recent studies [13].
Receptor-independent actions relate mainly to the antioxidant properties of melatonin and its metabolites which are able to scavenge reactive oxygen species (ROS) and reactive nitrogen species. Both the receptor-mediated and receptor-independent effects of melatonin may happen simultaneously [14].
Immune-hematopoietic system
Physiologically, the distinction between the immune system and the hematopoietic system does not make much sense because their cellular and humoral components are largely overlapping. Rather, it seems reasonable to distinguish between the two main functions of the immune-hematopoietic system, that is hematopoiesis and immunity. Hematopoiesis is a remarkable and complex mechanism giving rise every day to billions of erythrocytes, myeloid cells, and lymphocytes. Hematopoietic stem cells (HSCs) in the BM are responsible for the continuous production of all types of blood cells. HSCs reside in complex BM structures called hematopoietic niches which provide a specialized cellular and humoral microenvironment controlling both HSCs self-renewal and cell migration from the BM to replenish the blood with mature immunocompetent cells. This vital process is regulated by the interaction of a variety of cells including sinusoidal endothelium, perivascular mesenchymal stromal cells (MSCs), regulatory T cells (Treg), macrophages, megakaryocytes, granulocytes, and osteoblasts [15–17]. Sympathetic nerves and non-myelinating Schwann cells also play an important regulatory role in the hematopoietic niche [18]. The formation of multipotent mesenchymal progenitors (MMPs) that lose the self-renewal ability is considered as the first step of HSCs differentiation. The second step is the differentiation of MMPs into common myeloid progenitors (CMPs) and common lymphoid progenitors (CLPs). Next, CMPs can give rise to granulocyte-macrophage progenitors (GMPs) and megakaryocyte-erythroid progenitors (MEPs). Finally, GMPs differentiate into monocytes, macrophages, mast cells, or granulocytes, and MEPs into erythrocytes and megakaryocytes. On the other hand, T and B lymphocytes, natural killer (NK) cells, dendritic cells (DCs), and the recently discovered innate lymphoid cells (ILCs) derive from CLPs [19–23].
The immune system is composed of primary and secondary lymphoid organs with the former type including the thymus and BM, and the latter involving lymph nodes and the spleen. The immune system has the vital function to protect the organisms against microbial pathogens, toxins, or abnormal cells like cancer cells by mounting antigen specific immune responses. The key feature enabling the immune system to perform this remarkable job is the capacity to discriminate self from non-self, based both on the ability of phagocytes to recognize pathogen-associated molecular patterns (PAMPs) and on the lymphocyte recognition of the proteins composing the major histocompatibility complex class I (MHC-I) expressed in all nucleated cells. Usually, a distinction is made between innate and acquired immunity although physiologically these two arms of the immune system are functionally interconnected. The first line of defense against an invading pathogen is exerted by physico-chemical barriers provided by epithelia and their secretions (antimicrobial peptides, lysozyme, lactoferrin, and secretory immunoglobulin A antibodies). When these barriers fail and the pathogen enters the body, its PAMPs are immediately recognized by phagocytes (neutrophils, monocytes, macrophages, and DCs) and by NK cells which eventually may destroy the invader or, in the case of macrophages and DCs, instruct the adaptive immune response by presenting its antigens in association with MHC-II molecules to B and T lymphocytes which respectively generate specific antibodies able to bind specific molecular structures of the pathogen and antigen specific cytotoxic T cells. The concerted action of these immune effectors kills the pathogen and eventually activates innate phagocytes that clear it from the organism. Finally, activated T and B lymphocytes differentiate in antigen specific memory cells conferring to the host a long lasting protective immunity against the microbial agent.
This review will focus on the features of the very complex circadian regulation of the immune-hematopoietic system which appear to hold a biological significance inspiring possible therapeutic approaches. The listing of the countless neuronal and hormonal effects on the various cellular components of the immune-hematopoietic system will be avoided.
Circadian rhythm regulation of hematopoiesis
The SCN conveys circadian information to peripheral organs through the sympathetic nervous system (SNS). In fact, clock gene expression is regulated by adrenergic mechanisms in a variety of mouse and human tissues including BM [24]. However, it has been also reported that peripheral circadian rhythms are maintained in dopamine β-hydroxylase knockout mice in which norepinephrine (NE) and epinephrine (E) cannot be synthesized [25].
The first evidence that the photoperiod could influence hematopoietic reconstitution after syngeneic BM transplantation in mice was provided by a study we published in 1992 [26]. We found that constant environmental lighting could inhibit leukocyte reconstitution after BM transplantation via adrenergic signals mediated by alpha1-adrenergic receptors. In addition, SNS inhibition by alpha1-adrenergic antagonists resulted in a myeloid-biased hematopoietic reconstitution at the expense of the lymphoid lineage. Further studies showed that alpha1-adrenergic receptors are expressed in a population of lymphoid/stem cells [18, 26]. This effect is consistent with recent findings suggesting the existence of myeloid-biased or lymphoid-biased HSCs, which beside the capability of reconstituting all blood cells show a predisposition to differentiate towards either myeloid or lymphoid lineage [27]. However, in addition to a direct circadian effect on hematopoiesis exerted by adrenergic agents in the hematopoietic niche, there is also an indirect influence by SCN-driven hormonal factors such as adrenal glucorticoids (GCs), melatonin, and inflammatory cytokines.
Direct adrenergic effects
Sympathetic nerves associated with blood vessels enter the BM parenchyma and by releasing catecholamines may influence HSC function. Circulating E and NE can also reach the hematopoietic niche. In addition, BM cells themselves as well as mature immunocompetent cells may synthesize catecholamines [28–30].
The first evidence that the NE concentration in murine BM varies according to a circadian rhythm was provided by my group in collaboration with Prof. Cosentino’s lab [31]. We found that the concentration of NE peaked during the night (the active phase in rodents) and correlated with the amount of cycling hematopoietic cells [31, 32]. These findings implied that the SNS conveys circadian information to the BM. Interestingly, we also found that exogenous NE could promote hematopoiesis in mice and protect the BM from the toxic effect of lethal doses of carboplatin [33]. We got evidence that the hematopoietic rescue was exerted on CMPs, nevertheless, further studies confirmed our findings at the HSC level in both mice and humans [34, 35]. Consistent with the inverted activity phases of the two species (night in mice and day in humans), circulating murine and human leukocytes cells show an opposite circadian rhythm even when they are present together in a model of humanized mice indicating the existence of a species-specific clockwork mediating the neural signals [36].
Together with mature leukocytes, hematopoietic stem and progenitor cells (HSPCs) also migrate from the BM to the blood circulation and back to the BM again (HSPCs homing) in a circadian fashion [37]. Variation in the expression of the chemokine C-X-C motif ligand 12 (CXCL12) in the BM is crucial in determining such traffic. In mice, light induced noradrenergic signaling downregulates CXCL12 by activating beta3-adrenoceptors on nestin+ MSCs. This promotes the HSPC migration from the BM into the blood. At night the opposite happens, the production of CXCL12 increases and HSPCs return to the BM [36]. The beta3-adrenoceptors’ role in HSPC mobilization is helped by beta2-adrenoceptors that may regulate clock genes expression in BM MSCs [38]. Similarly, the circadian leukocyte adhesion to vascular endothelial cells and migration to mouse tissues and BM during the night was suggested to depend on beta2-adrenoceptors stimulation. Thus, the SNS plays a central role in regulating the circadian mobilization of leukocytes and HSPCs [39]. In addition, the circadian oscillation of the SNS activity might well control the ratio of lymphoid vs. myeloid proliferation and differentiation via alpha1-adrenergic receptors as suggested by our study showing that hematopoietic reconstitution after syngeneic BM transplantation in mice kept under a 12 light/12 dark photoperiod was biased toward the myeloid lineages [26]. However, this important issue deserves further investigation.
Indirect hormonal effects
In steady state, the SCN rhythmic activity provokes the PVN release of corticotropin-releasing hormone which in turn stimulates adrenocorticotropic hormone production by the anterior hypophysis. This finally results in the production of GCs by the adrenal cortex [40]. As a consequence, in diurnal animals the blood concentration of GCs peaks in the early morning while in nocturnal species this happens at the beginning of the darkness period. In the BM, GCs play an important role in regulating HSC mobilization by acting on hematopoietic cells. However, this effect is exerted only within the physiological range of GC concentrations as high GC doses inhibit HSC mobilization [41]. In addition, the circadian GC oscillation may support HSPC proliferation by Notch1 signaling [42].
The biochemical messenger of darkness melatonin is also implicated in the regulation of BM functions. Exogenous melatonin could rescue hematopoiesis from the toxic effect of cancer chemotherapy compounds in cancer bearing mice. This effect was apparently mediated by granulocyte/macrophage-colony stimulating factor whose production was stimulated by melatonin-induced opioid agonists binding to k-opioid receptors expressed on BM macrophages and interleukin 1 (IL-1) secretion [43, 44]. This finding was in line with preceding studies showing that opioid peptides may regulate IL-1 secretion by BM macrophages [45, 46]. Circulating melatonin from the pineal gland has also been reported to drive a circadian rhythm in the proliferation of colony forming units for granulocyte and macrophage [47].
Like catecholamines that may be produced in situ, melatonin also can be synthesized directly by BM cells [11]. Interestingly, it has been demonstrated that light and darkness onset produce a short-term increase in the concentration of NE and of the inflammatory cytokine tumor necrosis factor (TNF) with NE being essential for the TNF surge [48, 49]. The light induced NE and TNF burst preceded HSPC mobilization and differentiation while that induced by darkness was lower in amplitude and produced an increase in melatonin concentration which in turn induced HSPC quiescence and retention. Melatonin concentration in the BM was three-fold higher than that in circulation suggesting that it was produced in situ. Thus, it appears that the same signal produces opposite effects according to the circadian time. The underlying mechanisms were suggested to include ROS, endothelial permeability, and the circadian expression of MT1 and MT2 in HSCs and/or MSCs. In addition, the melatonin retention signal may include also the stimulation of osteoblast differentiation. Last but not least, the effect of melatonin seems to be the same in both human and murine HSCs [49]. Beside melatonin, the cholinergic system also seems to constrain the circadian HSPCs and leukocyte mobilization by its inhibitory action on the sympathetic tone together with its anti-inflammatory effect [50]. It is not known whether the parasympathetic circadian rhythm is driven by a central clock like the SCN.
An important component reinforcing the circadian machinery is provided by the fact that even the expression of adrenergic receptors, melatonin receptors, and the CXCL12 receptor C-X-C chemokine receptor 4 (CXCR4) shows circadian oscillations synchronized with the light/dark cycle [24].
The findings reported above clearly indicate that the hematopoietic niche is regulated by circadian rhythms. This phenomenon can be viewed from a general point of view as a basic feature of all adaptive systems of the body in a changing environment. However, the details of its biological benefit are still not completely clear. One of the main factors hampering a clear understanding of the biological function of HSC circulation is the fact that the number of HSCs in the blood is too low at any time of the day to allow a productive experimental approach. Therefore, different hypotheses have been formulated. As bone formation and hematopoiesis have in common many cellular and molecular players, it has been proposed that their circadian regulation reflects the homeostatic need to control the bone as a whole organ including the BM [24]. Another hypothesis views HSC migration and circulation during the resting phase of the day as necessary to regenerate the BM niche and/or to provide a pool of circulating progenitor cells to cope with possible environmental stress like inflammatory or immune responses [24]. In my opinion, this view is supported by the observation that both innate and adaptive immune responses show clear circadian oscillations that seem functional for equilibrated protection against invading pathogens (see below).
Circadian rhythms and immunity
Both innate and adaptive immune responses show a circadian oscillation. As for hematopoiesis we can distinguish between effects mediated directly by catecholamines either circulating in the blood or released by sympathetic nerve terminals in peripheral tissues and lymphoid organs, from those mediated by GCs and melatonin.
Direct adrenergic effects
A factor that may directly influence the magnitude of the innate inflammatory response in tissues is the circulation of myeloid cells in the blood and their infiltration in peripheral tissues. In general, circulating cells peak in the blood during the resting phase while their migration in peripheral tissues occurs during the active phase. At variance, human gamma delta (GD) T cells and CD8+ T cells peak in the blood during daytime and express more adrenergic receptors in comparison with other immune cell types [24]. However, virtually all innate immune cells do express a variety of adrenoceptors subtypes whose activation by catecholamines may modulate their functions [51]. Broadly speaking, beta-adrenergic activation dampens the inflammatory response while alpha-adrenoceptors activation has the opposite effect [51, 52]. Therefore, the effect of the SNS circadian rhythm on innate immune cells depends on their adrenoceptor repertoire. In addition, E and NE show a very different affinity for both alpha- and beta-adrenergic receptors, and their effects may thus be very different according to the target cell type and time of exposure to the adrenergic agonists [53]. Furthermore, catecholamines have also been reported to influence adhesion molecule expression and transendothelial cell migration of mature leukocytes [54]. Studies using local surgical sympathetic denervation in murine peripheral tissues showed that, in steady state, maintenance of the circadian rhythm of vascular leukocytes adhesion and tissue infiltration requires a functional innervation [54]. It has been suggested that the circadian control of the innate response is required to optimize its effect against an invading pathogen and that its disruption has negative consequences [55, 56]. However, the innate response is elicited not only by infectious agents but also in case of tissue injury associated with pathological conditions such as atherosclerosis, myocardial infarction, and ischemic stroke where similarly show a circadian oscillation [57].
A clear understanding of the local circadian adrenergic influence on migrating leukocyte function is hampered by their traffic in and out of the blood circulation and by their consequent periodic exposure to a variety of neuronal and hormonal factors. On the contrary, tissue resident macrophages and DCs provide a simpler model to study the effect of locally released catecholamines. Resident macrophages are a very heterogenous cell population involved in the maintenance of tissue homeostasis. Together with DCs, they have the function of immune sentinels by sensing PAMPs and have the unique role of linking the innate response with the adaptive immune response by inducing T cell differentiation into T helper (Th) cell effectors [58]. We reported that epidermal DCs (Langherans cells) migrate in response to NE and that the extent of Th1 cell differentiation is influenced by the local SNS activity in the early phase of their stimulation [59, 60]. In particular, it was found that activation of beta2-adrenoceptors resulted in reduced antigen-presenting ability and that the type of inflammatory reaction to contact hypersensitivity agents in the skin may alter local NE turnover and hence dictate the type of Th immune response elicited [60]. These findings have been basically confirmed by other studies [61, 62].
Consistently, a recent study shows that the induction of anti-tumor CD8+ T cells by DCs follows a circadian rhythm [63]. This interesting and therapeutically relevant effect depended on the circadian expression of the co-stimulatory molecule CD80 in the DCs present at the site of tumor inoculation in mice [63]. The most efficient anti-tumor immune response was induced in the late afternoon (i.e. at the end of the resting phase in mice kept under a 12 light/12 dark cycle). Furthermore, inverting the light/dark cycle also inverted the phenomenon suggesting that light could entrain this effect. In spite of this observation [63], the authors concluded that the effect observed was dependent on the autonomous circadian oscillation of Bmal1 clock gene in DCs that drove the circadian expression of CD80. Nonetheless, the entraining ability of light suggests the possible involvement of SCN-derived signals such as reduced SNS activity and/or reduced GC levels in the blood. Coherently, it has been reported that DCs show a circadian migratory pattern into draining lymph nodes depending on the circadian oscillation in the expression of the chemokine C-C motif chemokine ligand 21 (CCL21) that we have shown to be modulated by adrenergic agents [64, 65]. Similarly, another study reports that lymphocyte migration into lymph nodes peaked in phase with DCs in a circadian fashion at the beginning of the active period in rodents (night) that, as in humans is associated with activation of the SNS [66]. Lymphocytes and DCs are critical in mounting the adaptive immune response and their common behavior might be important for an efficient antigen presentation. Moreover, this oscillation in lymph node cellularity could influence the magnitude of the adaptive immune response in a model of experimental autoimmune encephalomyelitis. In analogy with the above mentioned DCs study [63], the authors used specific ablation of circadian clock genes in T cells and found that the circadian rhythm of lymphocyte migration into and egress from lymph nodes depended on both lymphocyte clock genes and environmental factors, yet they concluded that the effects observed depended by the autonomous oscillation of clock genes in lymphocytes [66]. Perhaps for the sake of cleanness, they did not take into consideration the cyclical release of NE by nerve varicosities as a component of the circadian machinery nor other possible indirect effects driven by the SCN.
In any case, the magnitude of both T and B cell responses varies according to the time of the day and this might be exploited to achieve a stronger protective response in case of vaccination.
Indirect hormonal effects
GCs are involved in the circadian regulation of innate immunity. The GC action involves in part clock gene expression that regulates their synthesis. During the active phase of the photoperiod, their anti-inflammatory and immunosuppressive effects may be indirectly exerted on innate immune cells by downregulating inflammatory cytokines production or chemokines production in other cell types [67, 68]. For example, the production of the chemokine CXCL5 by pulmonary epithelial cells that drives the migration of neutrophils and monocytes into the inflammatory site is controlled by the circadian production of GCs. As a consequence, also the local production of inflammatory cytokines and the whole response by the infiltrating cells follow a circadian rhythm [69]. GCs exert their anti-inflammatory effect by inhibiting nuclear factor kappa B (NF-kB) and mitogen-activated protein kinase (MAPK) pathways.
However, GCs do not exert only a suppressive effect on immunity but they may also promote the proliferation of T cells, B cells, and ILCs by enhancing the expression of IL-7 receptor alpha-chain. This produces an accumulation of T cells in secondary lymphoid tissues such as lymph nodes and spleen inducing a stronger immune response by effector CD8+ and CD4 T cells [70]. Thus, GCs seem to collaborate with adrenergic signals to maximize the immune response during the active phase of the photoperiod when the infection risk increases.
Another important circadian regulator of immunity is melatonin. Being the biochemical messengers of darkness, indoleamine has the important role of transducing the entraining effect of light both at the central level in the SCN and at the peripheral level in the immune system. Pinealectomy in various animal models has been associated with profound alterations of the immune system and its reactivity to antigenic challenges [71]. However, it has been our report in the 1980s that first demonstrated that melatonin may directly modulate the immune response [72]. Today, the immunoregulatory role of melatonin is widely recognized to the extent that the indoleamine or its agonists have been proposed for the treatment of viral, bacterial, and parasitic infections as well as autoimmune disorders [73–75]. Most interesting, it has been reported that melatonin may be of therapeutic value in sepsis and in coronavirus disease 2019 (COVID-19) [76–78]. The immunoregulatory effects of melatonin are possibly exerted at various levels. One of these might well be the direct effect of melatonin on immunocompetent cells as virtually all cells of the immune system express melatonin receptors [79]. Melatonin may stimulate inflammatory cytokine production or in certain conditions exert the opposite effect as well as stimulate anti-inflammatory mediators acting as an equilibrating agent on the immune response [80, 81]. In addition, as the circadian clock is involved in the regulation of the immune response against microbial pathogens and infections per se may disrupt the circadian expression of clock genes, melatonin might restore the circadian oscillation of immune effectors and thus help the immune system to overcome the disease [73]. Yet, if this effect depends also on a direct action of circulating melatonin on clock gene function in immune cells or if it is just mediated by its synchronizing action at the central level remains to be established. In addition, inflammatory cytokines released during the early phase of infection may inhibit melatonin synthesis in the pineal gland while increasing local melatonin production by inflammatory cells like macrophages [82]. This mechanism has been suggested to be the expression of an immune-pineal axis aimed at optimizing the response against invading pathogens and is consonant with the observation that infections may disrupt the circadian clock [73]. Both the production of extra-pineal melatonin at the site of infection and the inhibition of pineal melatonin synthesis seem to be aimed at maintaining the inflammatory response within safe limits suggesting an opposite action of circadian pineal melatonin vs. immune-derived melatonin.
Conclusions
The immune-hematopoietic system is under circadian control both in steady state or during its activation by environmental factors. As far as it concerns hematopoiesis, such a control is essential for HSCs, HSPCs, and mature leukocytes circulation as well as for their homing in the BM or peripheral tissues respectively. This cellular traffic is functional to maintain an efficient hematopoietic niche as well as to patrol the organism to ensure tissue homeostasis and an appropriate immunological reaction in case of infection. As the circadian control of HSC circulation is effective also when they are mobilized by colony stimulating factors [83], a higher number of HSCs could be harvested by apheresis if it were performed in humans at the beginning of the resting phase rather than in the morning. The number of HSCs is crucial for the success of HSC transplantation, thus this “circadian” procedure could be very useful. Moreover, as the main player governing HSC circulation is the SNS, the use of adrenergic agents to enhance the effect of colony stimulating factors should be investigated.
On the contrary, it is possible that the engraftment of human HSCs in the BM would be more efficient in the morning. HSC transplantation is the only therapeutic option in most hematopoietic disorders. However, the necessary elimination of the host hematopoietic cells is accompanied by a destruction of the sinusoidal network which is needed to ensure a long-term reconstitution of hematopoiesis. It has been reported that a key player driving the regeneration of the sinusoidal network and hence of hematopoiesis is the circadian production and subsequent elimination of neutrophils [17]. These cells together with other immune cells are crucial regulators of the hematopoietic niche [17]. As reported above, we have shown that inhibition of alpha1-adrenoceptors after syngeneic BM transplantation in mice resulted in an accelerated hematopoietic reconstitution in particular of the myeloid lineage obviously including polymorphonuclear cells [26]. Nowadays, highly selective ligands for alpha1-adrenoceptors are available [84], hence the role of alpha1-adrenoceptors mediated circadian signaling in the hematopoietic reconstitution after HSC transplantation would deserve to be investigated at the clinical level.
Modern lifestyles which alternate irregular patterns of activity, resting (sleep), and light exposure are responsible for an increased susceptibility to cardiovascular disorders, various types of cancer, and other diseases. This has been related to the disruption of circadian rhythms which in turn affects metabolic and immune parameters [85, 86]. In particular, leukemia development deriving from an uncontrolled proliferation of HSCs has been associated with a dysregulation of the circadian clock [87, 88]. Interestingly, it has been also reported that SNS blockade in the BM promotes acute myeloid leukemia by altering the hematopoietic niche [89]. Attempts to restore the SNS signaling by beta-adrenergic agonists showed an inhibitory effect on leukemic cell proliferation [89]. This approach, i.e. the use of adrenergic agents to modulate hematopoiesis in normal and pathological situations, would deserve to be more broadly pursued. Likewise, in spite of that dysregulated circadian rhythm has been recognized in a variety of disease states, trials aimed at restoring the circadian machinery are based only on bright light exposure and/or administration of exogenous melatonin [90]. This might well depend on our poor understanding of the hierarchical mechanisms governing the circadian clock, especially those linking the central clock to the peripheral ones. In fact, on one hand, it is well known how light may synchronize the SCN (Figure 1) and also at the cellular level the complex network of clock genes has been quite elucidated [91] but, on the other hand, how the two clock systems communicate each other is still not completely understood. It is, in fact, possible that beside the neuronal and hormonal signaling from the SCN to the periphery, core clock genes such as Bmal1 and Clock in immune-hematopoietic cells might feedback to central structures like the pineal gland. This might be achieved by influencing the circadian oscillation of inflammatory cytokine production as well as of Toll-like receptor (TLR) expression, a family of pattern recognition receptors (PRRs), and, finally, of the innate immune response [92].
One of the vital behavioral cues linked to the resting phase of the day is sleep. Sleep is crucial for a healthy immune system and vice versa immune-derived factors are needed for regular sleep. In addition, the sleep-wake or activity-rest cycle together with the oscillation in body temperature are the most evident and readily appreciable expression of the circadian regulation of the body [93]. The circadian release of inflammatory cytokines such as IL-6 and TNF-α plays an important role in sleep regulation [94]. Thus, the widely known sleep facilitating action of melatonin might depend also on its effect on inflammatory cytokine production. This would imply that the effect of melatonin on sleep might change according to the immune status of the host, a hypothesis easily verifiable at the clinical level. On the other hand, the disrupted circadian rhythmicity associated with a variety of diseases [90] might depend on the amount and quality of circulating inflammatory cytokines associated with the peripheral production of melatonin and/or catecholamines by immune-hematopoietic cells, in analogy with what is happening during the first phase of the immune response to an invading pathogen [82].
The possible interactions of the circadian regulation of the immune-hematopoietic system with sleep in steady state vs. a pathological condition are summarized in Figure 2. However, to what extent the disruption of circadian rhythmicity during, for example, the initial phase of infection represents a physiological response aimed at combating the invading pathogen or instead reflects a pathogenic phenomenon is still not clear. In other words, it is still obscure which mechanism activates the gradual recuperation of the circadian rhythmicity that is needed for full recovery from a disease. This issue is relevant to design the most appropriate therapeutic approach to restore disrupted circadian rhythms eventually associated with a pathological situation.
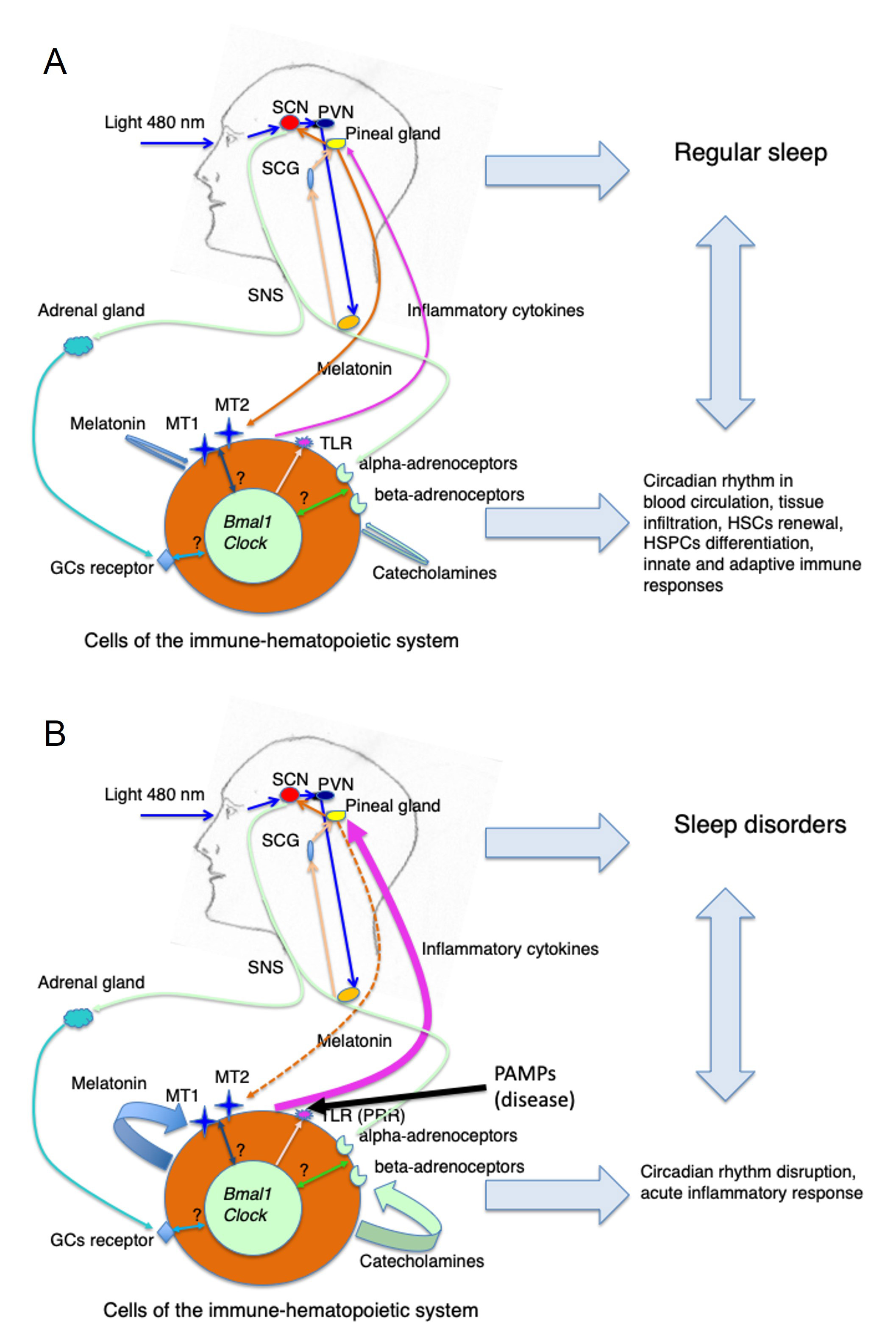
Circadian regulation of the immune-hematopoietic system in health and disease conditions. (A) Steady state (health): blue light (460–480 nm) entrains the SCN via the circadian production of pineal melatonin. The SCN drives the oscillation of the SNS activity that determines the circadian secretion of catecholamines activating beta- and alpha-adrenoceptors on cells of the immune-hematopoietic system. Amid a variety of effects on specific cell functions depending on the cell type and its adrenoceptors repertoire the SCN neuronal signaling might synchronize peripheral clock genes that in turn will determine the cyclical expression of receptors such as TLRs, MT1 and MT2, and GC receptors. Similarly, the circadian production of pineal melatonin and adrenal GCs contributes to the modulation of cell functions and perhaps to peripheral clock genes synchronization. The resulting circadian regulation of HSPCs and hematopoietic cell circulation, proliferation and differentiation, tissue infiltration, and of innate and adaptive immune reactivity contribute to maintaining a normal sleep pattern; (B) disease: upon activation of TLR (PRR) by PAMPs due to microbial infection or other pathological situations associated with an inflammatory response (autoimmune diseases, cancer, tissue injury) possibly inducing a local massive production and release of inflammatory cytokines as well as of melatonin and/or catecholamines by cells of the immune-hematopoietic system, the circadian machinery is eventually disrupted both at the pineal and peripheral level influencing innate and adaptive immune responses as well as sleep patterns. Whether this alteration in the circadian machinery has to be considered a physiological response aimed at combating the disease (i.e. immune-pineal axis) or, on the contrary, it should be considered only a pathogenic effect remains to be clearly established. In any case, the recovery from a disease state is associated with a restoration of the circadian rhythm and normal sleep pattern
The evidence that the immune-hematopoietic system is under circadian control is robust and offers a number of novel therapeutic approaches in the domain of chronotherapy. Vaccines and therapeutic interventions in case of infectious diseases and cancer should be administered taking into consideration the circadian oscillation of the immune response. In the same way, the management of autoimmune diseases might benefit from a similar methodology. In particular, vaccines and cancer immunotherapy should be administered during the peak of the circadian migration of DCs and lymphocytes (late at night in humans). The same schedule might be used for systemic immunosuppressive treatments of organ-specific autoimmune diseases. In addition, as immunocompetent cell migration and homing are governed by adrenergic mechanisms, a potential therapeutic approach might consider the combination of adrenergic agents with vaccines or other immunotherapeutic interventions. However, to date, the only chronotherapeutic approach widely investigated is the evening administration of exogenous melatonin. Being a multifunctional molecule, melatonin may indeed exert a myriad of effects. However, only a few of them may be, at least in part, related to its ability to restore the circadian rhythm as in sleep disorders [95] and cancer [96]. The bulk of its functions seems to depend on receptor-mediated direct action on peripheral targets or on receptor-independent effects mainly related to its detoxifying antioxidant properties. On the other hand, indoleamine may be produced endogenously by hematopoietic cells themselves [11], and this fact does not help our understanding of its immune-hematopoietic role. Perhaps this is also the reason why, apart from its protective action against the hematopoietic toxicity of cancer chemotherapeutic compounds [28, 96], the hematopoietic role of melatonin is still rather ambiguous. Similarly, the fact that hematopoietic cells may also synthesize and secrete catecholamines [29, 30], is another confounding factor in the comprehension of the immune-hematopoietic role of the circadian oscillation of the SNS activity. Finally, if the recently reported evidence that melatonin synthesis in DCs and macrophages may be triggered by beta-adrenergic receptors is added to this picture [97], the conclusion is that a complete understanding of the circadian modulation of the immune-hematopoietic system in health and disease is rather far away.
Abbreviations
BM: |
bone marrow |
Bmal1 : |
brain and muscle ARNT-like 1 |
Clock : |
circadian locomotor output cycles kaput |
CMPs: |
common myeloid progenitors |
CXCL12: |
C-X-C motif ligand 12 |
DCs: |
dendritic cells |
E: |
epinephrine |
GCs: |
glucorticoids |
HSCs: |
hematopoietic stem cells |
HSPCs: |
hematopoietic stem and progenitor cells |
IL-1: |
interleukin 1 |
MSCs: |
mesenchymal stromal cells |
MT1: |
melatonin type 1 receptor |
NE: |
norepinephrine |
PAMPs: |
pathogen-associated molecular patterns |
PRRs: |
pattern recognition receptors |
PVN: |
paraventricular nucleus |
SCG: |
superior cervical ganglia |
SCN: |
suprachiasmatic nucleus |
SNS: |
sympathetic nervous system |
Th: |
T helper |
TLR: |
Toll-like receptor |
TNF: |
tumor necrosis factor |
Declarations
Author contributions
GM: Conceptualization, Data curation, Formal analysis, Investigation, Methodology, Resources, Software, Validation, Visualization, Writing—original draft, Writing—review & editing.
Conflicts of interest
The author declares that there are no conflicts of interest.
Ethical approval
Not applicable.
Consent to participate
Not applicable.
Consent to publication
Not applicable.
Availability of data and materials
Not applicable.
Funding
Not applicable.
Copyright
© The Author(s) 2023.