Abstract
T cells play a central role in anti-tumor immunity, and reactive oxygen species (ROS) lie at the crossroad on the anti-tumor T cell responses. To activate efficient T cell immunity, a moderate level of ROS is needed, however, excessive ROS would cause toxicity to the T cells, because the improper level leads to the formation and maintenance of an immunosuppressive tumor microenvironment. Up to date, strategies that modulate ROS, either increasing or decreasing, have been widely investigated. Some of them are utilized in anti-tumor therapies, showing inevitable impacts on the anti-tumor T cell immunity with both obverse and reverse sides. Herein, the impacts of ROS-increasing and ROS-decreasing treatments on the T cell responses in the tumor microenvironment are reviewed and discussed. At the same time, outcomes of combination immunotherapies are introduced to put forward inspirations to unleash the potential of immunotherapies.
Keywords
Reactive oxygen species, T cells, tumor, therapy, immunotherapyIntroduction
Cancer immunotherapy refers to the therapeutic strategy that activates autologous anti-tumor immune responses to combat tumor cells [1]. Host CD8+ T cells mediate the recognition and cytotoxicity to malignant cells [2], and the presence of activated CD8+ T cells within the tumor mass has shown the significant positive prognostic import [3], which strongly indicates the central role of CD8+ T cells in the anti-tumor immunity. Currently available immunotherapies, including cytokines, vaccines, adoptive T cell (ATC) therapy, and immune checkpoint inhibitors, are all aiming to activate T cells through various ways [4]. However, the relatively low response rate and serious adverse events of immunotherapies are two shortcomings restricting their application [5, 6], and a great of efforts have been made to improve the response and avoid side effects.
An effective immunotherapy can promote the killing of tumor cells by the active T cell immunity [7], which is under the regulation of reactive oxygen species (ROS) [8–10]. ROS are small short-live oxygen-containing molecules that are chemically highly reactive and play a dual role in tumorigenesis and progression. Excessive amounts of ROS are often generated in the tumor microenvironment, which causes oxidative stress [11]. The intracellular ROS are generated mainly through nicotinamide adenine dinucleotide phosphate (NADPH) oxidases (NOX) and the respiratory chain, and can also be generated through exogenous stimulations including ultraviolet and radiation, air pollutants, and chemicals. Accumulated evidence has demonstrated that ROS are one double-edged sword, playing different roles in the T cell responses, for its participation in T cells activation process and in the formation and maintenance of immunosuppression as well. Therefore, tuning ROS would be an important strategy to improve the expected responses and reduce the side effects of T cell-based immunotherapies. The roles of ROS in the T cell immune responses, as well as the impacts of ROS-modulating reagents on the T cell immune responses and outcomes of T cell-based immunotherapies are reviewed and discussed in this article.
ROS in anti-tumor T cell immunity
An efficient anti-tumor immunity can be realized by the following steps. First, dendritic cells (DCs) capture and process tumor-associated or tumor-specific antigens and present to resident CD8+ T cells in the lymph nodes; and next, the CD8+ T cells are activated and migrate to the tumor site to recognize and destroy the tumor cells expressing specific antigens [12]. However, coexistence of tumor cells and T cells is often observed in some patients, indicating that the immunogenic tumor tissues are protected from immune attacks [7]. Up to date, it has been well documented that T cell immune responses in the tumor site can be profoundly suppressed and the T cells exhibit dramatic changes in the T cell receptor (TCR) signaling, proliferation and functions, resulting in the tumor immune escape [13, 14].
Over the past decades, tremendous progresses have been achieved in the understandings of how tumor cells evade the immune system (Figure 1). The major mechanisms by which tumors evade the destruction by the immunological system include (1) downmodulation of components of antigen processing and presentation machinery; (2) recruitment and education of suppressor immunological and stromal cells, such as regulatory T (Treg) cells, myeloid-derived suppressor cells (MDSCs), tumor-associated macrophages (TAMs), tumor-associated neutrophils (TANs), tumor-associated fibroblasts (TAFs), and vascular endothelial cells; (3) production of soluble factors associated with immunosuppression, such as transforming growth factor beta (TGF-β) and interleukin-10 (IL-10); and (4) upregulation of ligands for coinhibitory receptors that downmodulate the activity of tumor-infiltrated lymphocytes, such as programmed death ligand-1 (PD-L1) [15].
Interestingly, ROS lie at the crossroad of anti-tumor T cell immunity. As it is known that ROS signals are needed in the process of an efficient T cell immunity [9, 16]. Firstly, ROS participate in the processing and presenting of antigens by DCs. When DCs endocytose tumor-derived microparticles to lysosomes, the expression of NOX2 in the lysosomes is up-regulated to generate ROS [17]. DCs continuously produce ROS through NOX2 for at least 10 h after antigen uptake [18], and the sustained production of ROS causes maintained alkalinization of the phagosomal lumen. DCs those lack NOX2 show enhanced phagosomal acidification and increased antigen degradation, resulting in the impaired antigen presentation [19]. Afterward, when antigens are presented to T cells through DCs, TCRs on the surface of T cells are activated by the antigens. The TCR stimulation can induce a rapid production of ROS [20], leading to the activation and proliferation of T cells [21–23]. Furthermore, excessive ROS that are generated by some chemo-reagents, radiation, or photodynamic therapy (PDT) would induce immunogenic cell death (ICD) of tumor cells, and the released immunogenic molecules such as high-mobility group box 1 protein (HMGB1), ATP, and damage-associated molecular patterns (DAMPs) can activate the immunity of DCs and T cells [24, 25].
However, excessive ROS is closely associated with the suppression of T cell immunity [10]. A high level of ROS is related to the apoptosis and impairment of T cells. Activation-induced cell death (AICD) is a complex immunoregulatory mechanism that causes the demise of a fraction of T cells upon antigen-driven activation, a panel of proapoptotic factors in the tumor microenvironment induce AICD in tumor-infiltrating T cells, which contributes to the immune evasion of the tumor [26]. The induction of AICD of T cells is mediated by hyperpolarization of mitochondria and oxidative stress [26, 27]. Large amounts of ROS generation due to the downregulated mitochondrial superoxide dismutase 2 (SOD2) also impairs tumor-infiltrated T cells, and scavengers that neutralize mitochondrial ROS can partially restore CD8+ T cells function [28]. Furthermore, the formation and maintenance of CD8+ memory T cells need high levels of glutathione (GSH) generation through the pentose phosphate pathway to keep a low ROS state, however, the abrogation of the pentose phosphate pathway increases the ROS level, leading to the impairment of memory CD8+ T formation and maintenance [26, 29].
Excessive ROS also suppress T cell immunity by affecting other players within the tumor microenvironment that participate in the anti-tumor T cell responses. For example, Treg cells, which are indispensable for preventing autoimmunity by braking on the T cell immunity, can suppress the effective tumor immunity. ROS also participate in the differentiation of Treg cells [30], and mediate tumor immunosuppression through triggering SUMO-specific protease 3 (SENP3) accumulation [31]. Myeloid cells including MDSCs and macrophages, are recruited to the tumor site and accumulate in the tumor microenvironment, and MDSCs are immature and immunosuppressive myeloid cells. It has been reported that ROS participate in the maintenance of the immature state of MDSCs [32]. In addition, tumor cell-derived ROS also promote the migration of macrophages and educate them to a pro-tumoral M2 phenotype through phosphatidylinositol-3-kinase (PI3K) signaling [33]. These myeloid cells contribute to the high ROS levels triggering oxidative stress in the tumor microenvironment [12, 34], and also impair the T cell immunity through inhibiting TCR ζ-chain expression and desensitization of the TCR [35–37], suppressing the proliferation of T cells [38, 39], increasing expression of immune checkpoint [34, 35, 40], and releasing immunosuppressive cytokines like TGF-β and IL-10 [34, 41, 42]. Fibroblasts can be trimmed by tumor cells to differentiate to pro-tumoral myofibroblasts in ROS relating pathways [43], and the inhibition of ROS generation can normalize the fibroblasts [44]. Myofibroblasts-containing tumors often have low levels of lymphocytes [45], due to the exclusion of T cells [46].
The major players that participate in anti-tumor T cell response and their interactions with ROS are summarized in Figure 2. Because of the dual effects of ROS on the T cell immune responses, ROS can be a double-edged sword in the anti-tumor T cell immunity. Therefore, different ROS-modulating patterns may have impacts on the anti-tumor T cell immune responses and anti-tumor outcomes.
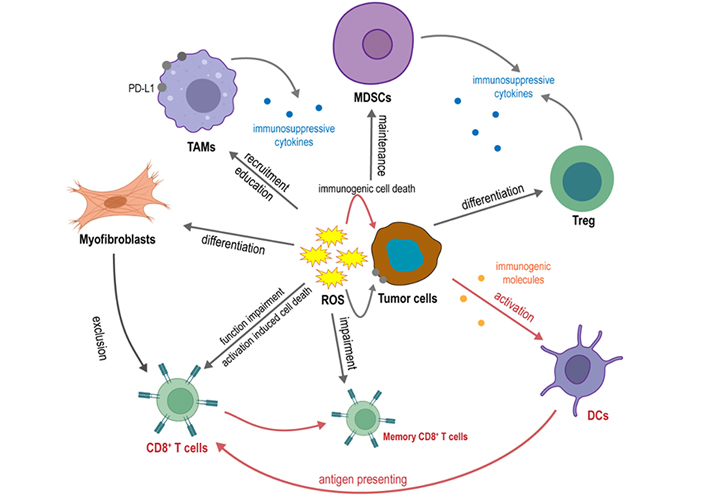
Major players that participate in anti-tumor T cell response and their interaction with ROS
Therapeutic strategies based on ROS-increasing and the impact on T cell immune responses
The induction of ROS leads to the preferential killing of tumor cells, therefore has been widely used in anti-tumor therapies. So far, various ROS-increasing therapeutic strategies have been developed, mainly including chemotherapy, radiotherapy, gas plasma therapy, photodynamic therapy, and sonodynamic therapy. It should be addressed that treatment-induced ROS have a dual effect, the ROS-increasing strategies and their impacts on the T cell responses and related profiles are discussed in this section.
Chemotherapy
Several chemotherapeutic drugs that are currently used for anti-tumor treatment lead to oxidative stress [47]. Chemotherapeutics disrupt normal endogenous ROS-generating routine and induce excessive superoxide radicals (O2•−) generation and derived hydrogen peroxide (H2O2) through impaired mitochondrial complexes [48]. There are studies reporting chemotherapy-induced ROS activates T cell responses in some cases while suppressing in others, possibly due to the dual effect of ROS on T cell immune response.
Chemotherapy-induced ROS augments T cell infiltration in the tumor microenvironment through both induction of ICD and release of chemokines. Bleomycin, an anti-tumor antibiotic glycopeptide and causes DNA breaking through oxidative stress [49], was reported to induce ICD by increasing the ROS level of the tumor cells. The dying cells increased surface exposure of chaperones and liberation of HMGB1 and ATP, driving CD8+ T cells dependent anti-tumor immunity [50]. Similarly, docetaxel induced ROS generation and HMGB1 release, which increased CD8+ T cells recruitment [51]. Besides, palbociclib, an inhibitor of cyclin dependent kinases 4 and 6 (CDK4/6), increased ROS along with the expression of C-C motif chemokine ligand 5 (CCL5), C-X-C motif chemokine ligand 9 (CXCL9), and CXCL10 in the triple-negative breast cancer (TNBC) cells and facilitated the recruitment of CD8+ T cells to the tumor site in TNBC bearing mice [52].
However, it can be noticed that pieces of literature also reported the immunosuppression effect of chemotherapy-induced ROS. For example, Bleomycin was also reported to induce the expansion of Treg cells via its capacity to induce TGF-β secretion by tumor cells [50]. Paclitaxel and buthionine sulphoximine generated excessive ROS in the tumor cells as well as in the macrophages, leading to the upregulation of PD-L1 and secretion of immunosuppressive cytokines IL-4, IL-10, and IL-17 in TAM both in vitro and in vivo [53]. These immunosuppressive cells, immune checkpoint molecules, and chemokines compose the unfavorable face of ROS-induced chemotherapies.
In summary, chemotherapy-induced ROS in tumor cells can kill tumor cells and activate T cell immune response through immunogenic contents release and recruitment of CD8+ T cells. But at the same time, massive ROS also lead to the formation and recruitment of immunosuppressive cells and cytokines. Selecting appropriate chemotherapeutic drugs, such as those that induce immunogenic death through ROS-independent pathways, may help to elude the immune suppression and improve benefits.
Radiotherapy
Ionizing radiation is widely used to treat many types of cancer. Ionizing radiation induces a substantial increase in ROS levels through photons generated from cobalt, cesium, or a linear accelerator or particles such as electrons, protons, neutrons, α-particles and β-particles. Radiation induces ionization of H2O into •OH upon exposure [54]. The transient but robust release of •OH after irradiation may oxidize biomolecules such as proteins or lipids in the cells and cause mitochondrial dysfunction [55]. O2•− is then generated from the leakage of electrons that is associated with the dysfunction of the electron transport chain (ETC). A decreased activity of mitochondrial ETC complexes has recently been found to increase the cellular H2O2 level [56]. Besides, NOXs can also be activated by radiation exposure, leading to persistent generation of O2•− [57, 58]. Therefore, transient •OH and persistent O2•− and H2O2 are major ROS involved in radiotherapy.
It has been recognized that the radiation also interferes with T cell immune responses. Radiation therapy increased cytotoxic T cells (CTLs) population both in the tumor site of Lewis lung carcinoma (LLC) subcutaneously implanted in mice [59] and the peripheral blood mononuclear cells (PBMC) of primary nasopharyngeal carcinoma patients [60]. However, like chemotherapies, radiation was also revealed to upregulate the Treg cell population in primary nasopharyngeal carcinoma patients [60], which would suppress T cell responses. In addition, the radiation treatment induces injury of normal tissues through ROS, which also has significant impacts on the progress of radiotherapy and the survival and prognosis of patients [61], therefore, pre-supplement of dietary antioxidants sometimes was used to provide radiation protection in normal tissue [62]. Nevertheless, the effects of pre-supplement of dietary antioxidants on anti-tumor immunity are still pending, antioxidants might decrease the Treg cell population, but at the same time, calm down the CTLs. In this aspect, antioxidants should be applied with caution.
Photodynamic and sonodynamic therapy
PDT relies on the photodynamic action, which is an oxygen-dependent reaction following photosensitization. PDT has evolved into a safe and effective dermatologic treatment option and expanded outside of the field of dermatology to solid tumors over the past decades [63, 64]. However, PDT cannot penetrate deep tissues to kill the tumor cells. To overcome this limit, sonodynamic therapy (SDT) employing ultrasound and sonosensitizers has been developed [65, 66]. In the presence of oxygen, the photoactivated photosensitizers or ultrasound-activated sonosensitizers lead to the formation of ROS. The major ROS generated in PDT and SDT is singlet oxygen (1O2), formed by the energy transfer from the triplet state of a photosensitizer. Radical species such as the O2•− and •OH can also be generated [67]. The generating mechanisms of these reactive species are stimulated by light irradiation, followed by their anti-tumor effect, and the synergetic principles with other therapeutic modalities could be found in a comprehensive review [68].
Recently, it is noticed that PDT and SDT can play roles in the modulation of T cell immune responses. The generated ROS are cytotoxic to the cells, and different cell death pathways are involved in the cytotoxicity of PDT and SDT, including necrosis and immunogenetic cell death, which stimulate the anti-tumor immunity [67]. Surface calreticulin expression and serum HMGB1 secretion were observed both in LLC cells in vitro and LLC tumors growing in mice [69]. The calreticulin expressed on the tumor cells then bound to the surface marker CD91 on DCs, stimulating them to grow into matured DCs, and inducing CD8+ T cell proliferation and infiltrating to the tumor site, therefore repressed the tumor growth and prolonged survival of a CT26 mouse model [70]. Local PDT treatment of tumors can control the growth of tumors outside the treatment field due to anti-tumor immune memory [71]. However, ROS generated in the tumor microenvironment can induce immunosuppression. The consumption of oxygen in the tumor microenvironment during the PDT process exacerbated the hypoxia, increasing portions of Treg cells and MDSCs in the tumor site in the PDT-treated TNBC mice [72]. The ROS generation upon PDT also stimulated interferon-gamma (IFN-γ) secretion, which could upregulate PD-L1 expression [73–75] and be harmful to the outcome.
Gas plasma
Atmospheric pressure gas plasmas are emerging as a promising treatment in cancer [76]. Plasmas were originally developed for low-temperature decontamination and wound healing, and were discovered to interact with organic materials without causing thermal/electric damage to the cell surface. This eventually led to the development of a wide range of reliable and user-friendly plasma sources that can deliver mild, yet effective doses of reactive species, and electromagnetic radiation to combat solid tumors [76]. Current progress of gas plasma in anti-tumor therapies has been summarized by a comprehensive review article [76].
Gas plasma can generate H2O2, 1O2, hypochlorous acid (HOCl), peroxyl (RO2•), alkoxyl (RO•), hydroperoxyl (HO2•) peroxynitrite (−ONOO) and •OH, depending on the feed gas and mode [77, 78]. Through generating ROS, gas plasma can induce ICD of tumor cells in the mice model of melanoma [78, 79] and breast cancer [80], therefore increasing DCs activation along with T cell infiltration, resulting in decreased tumor burden and prolonged survival. No antagonized effect was reported so far, indicating that gas plasma is a more ideal ICD-inducing strategy. The reason may be that the gas plasma can act on the tumor site more accurately than systemic chemotherapy and radiotherapy and does not consume oxygen compared with PDT, thus avoid to induce the formation of immunosuppression cells. It is also suggested that more efforts should be made to improve the targeting ability of the systemic ROS-increasing chemotherapy and radiotherapy.
Hyperbaric oxygen therapy
Hypoxia is a critical hallmark of solid tumors and involves enhanced cell survival, angiogenesis, glycolytic metabolism, and metastasis. Hyperbaric oxygen (HBO) is based on the administration of 100% oxygen at higher than normal atmospheric pressure, which can be used to overcome hypoxia and showed an inhibitory effect on some cancer types [81]. The HBO therapy can overcome the hypoxia in tumor tissues, therefore downregulating ROS levels in glioma cells and brain cells in the intracranial glioma mouse model [82]. However, thymus ROS levels were significantly up-regulated under the HBO stimulation, CD3+ T cells were decreased and Treg cells were increased both in the thymus and in the tumor tissues, resulting in T cell immunosuppression by inhibiting the T cells maturation, and promoting the growth of the transplanted GL261 glioma [82]. The HBO-induced ROS generation, as well as enhancement of Treg cells function, were also observed in psoriatic dermatitis and atopic dermatitis [83, 84]. These studies suggested that in the systemic treatment, the effects on the immune system should also be taken into consideration to evaluate the outcome of the therapy.
Given above together, ROS-generating therapies have obtained sustained attention both in the traditional therapies and in some newly developed therapies. Excess generation of ROS plays a dual role in anti-tumor T cell responses. The positive impacts include ICD and substantial DCs stimulation and CD8+ T cell activation and infiltration into the tumor site to kill tumor cells. However, excessive ROS also upregulates expression of PD-L1, increases the population of Treg cells and MDSCs, and induces thymus toxicity, which would suppress anti-tumor immune response, and prompt tumor progression.
Their hidden shortcomings on anti-tumor T cell responses should be taken seriously in the era of immunotherapy. Noted that among these therapies, the chemotherapy and HBO are systemically administrated and therefore may induce ROS generation systemically, which may be toxic to the immune organs. Therapies based on the irradiation, light, ultrasound, or plasma, are administrated to the tumor site and generate ROS locally accordingly. In these cases, the specificity should be improved to prevent damage to surrounding tissues due to the excessive ROS generation.
Therapeutic strategies based on ROS-decreasing and the impact on T cell immune responses
Because of the important roles of ROS in the progression, metastasis, and drug-resistance of the tumor, scavenging ROS has long been acknowledged as a therapeutic strategy [85]. Some clinical trials and pre-clinical studies considered that ROS-scavenging plays a positive role in anti-tumor therapy. However, the roles of ROS in T cell responses keeps controversial. According to the mechanisms, ROS-decreasing therapies can be divided into antioxidants, NOXs inhibitors, and metabolism regulators, and the three sorts of treatment and their impacts on the anti-tumor T cell immunity are discussed.
Antioxidants
It has been known that some nutrients have antioxidant activity, and some of them show in vitro or in vivo immune-modulating effects. Vitamins and their derivates are the most common antioxidative nutrients. Investigations have showed that Vitamin E supplement can modulate T cell responses both in human and in the mouse models. The high-dose supplement of Vitamin E enhances the secretion of IL-2 by the helper T cells of patients with advanced colorectal cancer, showing improved immune responses [86]. Vitamin E can also reduce the percentage of MDSCs in the tumor loci, reverse the suppression of T cell activation by MDSCs in TC-1 tumor-bearing mice, and therefore suppress the progression of the tumor [87].
All-trans retinoic acid (ATRA), a derivative of vitamin A, has been widely used in the treatment of several dermatological conditions [88] and has shown anti-tumor effects, especially on acute promyelocytic leukemia (APL) [89]. ATRA can decrease ROS through direct neutralizing or upregulating the expression of glutathione synthase, which leads to the accumulation of GSH [90–92]. In vitro investigation reveals that ATRA can promote the maturation of MDSCs and abrogate MDSCs-mediated immunosuppression, which improves T cell functions [90–92]. ATRA also upregulates the major histocompatibility complex (MHC) class II expression and costimulatory molecules in MDSCs separated from peripheral blood and therefore have the potentials to present antigens to T cells [93]. Due to the ROS-modulating ability and the abrogation of MDSCs-drive immunosuppression, the subcutaneous implantation of ATRA pellets by trocar injection at the side contralateral to the tumor strengthens the anti-tumor effects of tumor vaccines in several tumor-bearing mouse models, including 7,12-dimethylbenz(a)anthracene induced mammalian adenocarcinoma transfected with the hyaluronic acid molecule (DA3-HA tumor), C3 fibrosarcoma, methylcholanthrene-induced sarcoma [94]. However, although ATRA increased the ratio of myeloid/lymphoid DCs and improved the ability of patients’ mononuclear cells to stimulate T cells, it showed reverse outcomes with IL-2 therapy on patients with metastatic renal cell carcinoma [95].
Other than vitamins, components extracted from animals or plants also show antioxidative activity. Green tea is a natural source of antioxidant polyphenols (known as catechins) and epigallocatechin-3-gallate (EGCG). A green tea extract can decrease ROS in the CD34+ cells of bone marrow (BM) and increase the frequencies of total CD8+ T cells and CTLs cells in peripheral blood (PB), reduce Treg cells both in BM and in PB, therefore increasing the median survival of elder acute myeloid leukemia (AML) patients with myelodysplasia-related changes compared to the control cohort [96]. Another example is elemene, a sesquiterpene compound extracted from the rhizome of Curcuma herbs. Elemene shows a strong activity of scavenging ROS both in the tumor cells and in the macrophages, which prolongs the survival of TNBC mice [97]. Omega-3 polyunsaturated fatty acids, which act as enhancer factors in antioxidant defense against ROS, also show immune-modulating effects among patients with generalized solid tumors by increasing the ratio of T-helper cells to T-suppressor cells and restoring the tumour necrosis factor alpha (TNF-α) production in the malnourished cancer patients, and therefore prolonging the survival of patients [98, 99].
Some synthetic molecules or composites hold the ROS scavenging ability. 10-(6’-plastoquinonyl) decyltriphenylphosphonium (SkQ1) is an antioxidant with relatively high mitochondrion membrane penetrating ability and potent antioxidant capability which selectively scavenge mitochondria ROS [100]. The supplementation with SkQ1 in drinking water led to a decrease in the percentage of CD8+ T cells in the healthy mice [101]. Noted that in the orthotopic Panc02 implanted murine model of pancreatic ductal adenocarcinoma, the supplementation with SkQ1 in drinking water can modulate the immune cell composition, which may explain why the administration of SkQ1 inhibited angiogenesis, reduced metastases, though didn’t prolong the survival of the tumor-bearing mice [102]. An investigation of nanoplatform containing polypropylene sulfide and collagen-targeting peptide shows an effect of sweeping extracellular ROS in the tumor microenvironment in a murine model of 4T1 breast tumor and CT26 orthotopic colorectal cancer [103]. When combined with oleandrin, an anti-tumor drug that induces HMGB1 release, the nanoplatform can maintain the stimulatory activity of oleandrin to realize DCs activation and antigen processing to T cells with an effective anti-tumor immune response [103].
Nevertheless, one can notice that there have been conflicting reports on the role of antioxidants in tumor therapy for a long time. In some studies, antioxidant supplements prevented the tumor growth, on the contrary, other investigations reported these interventions are harmful to the patients [104]. For example, a mixture of selenium, vitamin E, and β-carotene supplements was reported to significantly reduce total cancer mortality [105]. But some ROS scavengers such as vitamin E, N-acetylcysteine (NAC), and Trolox, drive the metastasis of melanoma [106]. We would like to declare that the dual effect of antioxidants in anti-tumor T cell responses exists. In some models, antioxidants augment T cell responses and strengthen the anti-tumor outcome, while in other models, antioxidants weaken T cell responses and the overall outcomes. Immunosuppressive remission and T cell inactivation are the two ends of balance. The bi-directional effect of antioxidants on the anti-tumor T cell immunity may explain the conflicting role in the tumor therapies. Based on these findings, the systemical supplement of antioxidants should be more cautious in anti-tumor clinical applications and more efforts should be made in the development of targeted delivery of antioxidants to the tumor microenvironment, which need a fuller understanding of the tumor biology.
NOX inhibitors
NOX inhibitors are the most widely used ROS-decreasing reagents by inhibiting the generation of ROS. Several NOX inhibitors have been developed and used in the treatment of cardiovascular diseases, neurodegenerative disorders, and cancer [107, 108], and some of them are reported involved with T cell immunity-modulating effects.
A broad-spectrum NOX inhibitor, diphenylene iodonium (DPI), which shows inhibiting activity towards all isoforms of NOXs, can inhibit mononuclear phagocytes-derived ROS, and protect T cells from immunosuppression induced by the mononuclear phagocytes and increase CD69 expression in CD8+ T cells in an in vitro study [109]. Histamine, a naturally occurring pleiotropic bionic amine, well known for its role in inflammatory and allergic reactions, has shown anti-tumor activity in several malignancies [110–112]. Histamine can act as a NOX2 inhibitor to inhibit ROS generation in the tumor cells as well as in the tumor infiltrated cells [109]. By inhibiting ROS generation in MDSCs, histamine drive the cells to differentiate into DCs and reduce the accumulation of intratumoral MDSCs [113]. Therefore, histamine can increase the fraction of intratumoral CD8+ T cells with an effector phenotype owing to the abrogation of MDSC-induced suppression and enhancement of the antigen-presenting ability of DCs [114]. However, a recent study has revealed that histamine is increased in the tumor microenvironment, driving macrophages to polarize toward the M2 phenotype through the up-regulated histamine receptor H1, therefore rendering T cells dysfunctional through histamine receptor H1 (HRH1) [115]. The bi-direction effect of histamine depends on its dual physiological function: the NOX2 inhibiting activity is favorable to the anti-tumor T cell immunity, while the activation of histamine receptors is detrimental. To avoid the hidden side effect of histamine that is related to its intrinsic physiological function, specific small molecule inhibitors of NOX2 may be utilized in anti-tumor therapies. An increased surface expression of NOX4 is the biomarker of the differentiation of TAFs [116]. Setanaxib, a small molecule NOX4 inhibitor, inhibited ROS generation in TAFs, promoted intratumoral CD8+ T cell infiltration in TAF-rich tumors, therefore repressing tumor growth of TC-1 and MC-38 subcutaneously implanted mice [44, 117].
Given above together, the NOX inhibitors participate in the T cell immune responses including T cell infiltration, activation, and survival by reducing ROS generation within myeloid cells and TAFs. The above application of NOX inhibitors is based on the fact that NOX2 and NOX4 are specifically overexpressed in myeloid cells and TAFs, therefore, the effect of other NOX2 and NOX4 inhibitors on T cell immunity might resemble histamine and setanaxib. Moreover, the expression of the NOX family in immunosuppressive cells and T cells needs to be described thoroughly to optimize the usage of NOX inhibitors in tumor treatment.
Metabolism modulators
The generation of ROS can be regulated by mitochondrial energy metabolism [118], and some drugs decrease ROS generation through modulating metabolism. For example, metformin is a prescribed drug for the treatment of type 2 diabetes and has been reported to have anti-tumor effects through immune regulation. Metformin redirected the metabolism of CD11b+ cells (MDSCs and TAMs) to lower oxidative phosphorylation while elevating glycolysis, reducing the ROS production and proton leakage in the MDSCs and TAMs [119], which makes it able to help tumor-infiltrating CD8+ cells recover from the exhaustion [120], attenuate Treg cells differentiation in tumors through the activation of mTORC1 [121], and decrease PD-L1 expression on tumor cells [122]. By these actions, metformin is considered to abolish the suppressive effects on T cell responses, leading to the growth inhibition of several tumors in the syngeneic mouse models [119]. Methionine enkephalin, an opioid receptor agonist, acts as a significant mediator to connect the nervous system and the immune system [123]. In a murine model of CT26 colon carcinoma, methionine enkephalin reduces glycolysis and decreases ROS production in MDSCs, which promotes the MDSCs differentiation and increases CD8+ T cells infiltration in the lymphoid organs and tumor tissue, resulting in the enhanced immune responses in both tumor-bearing mice and cytoxan-induced immunosuppressive mice, and ultimately, inhibiting the tumor progression [124]. As4S4 is a mineral medicine usually used as one component of some formulations in the treatment of hematological malignancies [125]. When fabricated to hydrophilic nanocrystals, it can be internalized by the leukemia cells [126, 127] and murine breast cancer tumor cells [128], effectively eliminating the intracellular ROS through direct scavenging ROS and inhibiting the cellular respiration [126, 128, 129], leading to the suppression of inflammatory microenvironment in the TNBC mouse model, resulting in prolonged survival [128].
ROS can also be modulated by other distant targets. For example, embelin is a small molecule inhibitor of X-linked inhibitor of apoptosis (XIAP) and has been reported to inhibit ROS generation and impaired immunosuppression of MDSCs via limiting CCAAT enhancer binding protein beta (C/EBPβ) and signal transducer and activator of transcription 3 (STAT3) signaling [130]. The impairment of MDSCs contributes to the infiltration of CD8+ T cells and DCs and the depletion of Treg cells, leading to the restored T cell responses [130]. Besides, IPI-549, an inhibitor of PI3K, has been reported to downregulate ROS in the MDSCs and promote the apoptosis of MDSCs along with the proliferation and activation of CTLs [131]. The overall anti-tumor activity of these substances relies on their functional targets, meanwhile, their ROS-modulating effects show a surprising amicable effect on the anti-tumor immunity, and hostile effects have rarely been reported, demonstrating their possibilities to cooperate with immunotherapy.
In comparison, antioxidants as a monotherapy usually do not show remarkable outcomes, while the monotherapy of NOX inhibitors and metabolism regulators show anti-tumor activity in vivo. This could be explained by that small molecules of antioxidants cannot compete with the speed of ROS production, the reaction of superoxide with NO to form peroxynitrite occurs with a rate constant of (3.7 ± 1.1) × 107 L/(mol·s), which is too fast for any known antioxidant molecules to be effective [132]. Besides, antioxidants do not selectively scavenge ROS despite the type of ROS or the cells, which may bring the risk of calming down the activation of DCs and T cells, and NOXs inhibitors only exhibit their targetability to the differential expression profiles of NOXs in any cells. Hence, targeted administration of antioxidants and NOXs inhibitors to the tumor microenvironment may represent more sufficient regimens to strengthen the anti-tumor T cell responses.
Combination of ROS-modulating strategies in T-cell based immunotherapies
Combination therapy is a treatment modality that combines two or more therapeutic reagents and is helpful to overcome the limitations and toxicity of monotherapy. Throughout their lifetime, the patients are inevitably administrated with different treatments, ideally, these treatments work synergically to maximize the outcome. Efforts have been made to improve anti-tumor immune responses through virous strategies [133]. However, due to the dual role of ROS in the anti-tumor immunity as well as in other therapeutic reagents, ROS-modulating effects should be taken into consideration when applying the combination therapy.
As mentioned before, ROS can be regulated in two directions: increasing or decreasing. Radiotherapy, photodynamic therapy, sonodynamic therapy, and plasma therapy are typical ways of increasing ROS. The schemes to reduce ROS mainly include antioxidants, antioxidant enzymes, NOXs inhibition, and metabolic regulation. In terms of ROS-modulating sites, these treatments can be divided into two groups, systemic regulation and local regulation. The former includes chemo-agents, radiotherapy, hyperbaric oxygen therapy, dietary supplement, and the latter includes photodynamic therapy, sonodynamic therapy, and plasma therapy. In addition, some tumor-targeting strategies can help to transit systemic regulation to a local one. The on-targeting and off-targeting effects of both ROS-increasing and ROS-decreasing strategies are summarized in Figure 3.
It’s not hard to find out that the generation of ROS always obtains remarkable outcomes of destroying cancer cells but affects healthy tissues inevitably; while strategies that scavenge ROS are hardly used alone but often used as regulators and supplements. In the following section, the combination of immunotherapies with different ROS-regulating schemes will be introduced.
Cytokines
Cytokines are the first class of immunotherapy to be introduced into clinics, and injected cytokines can directly stimulate the growth and activity of immune cells [134]. The combination of ROS inhibitor histamine and IL-2 provides illumination for ROS-modulating immunotherapy. IL-2 has been used in the treatment of melanoma, renal carcinoma, and acute myeloid leukemia, and histamine as an adjuvant to IL-2 has been reported to be associated with a significant prolongation of survival compared with IL-2 alone in metastatic melanoma patients with liver involvement through ROS scavenging [111, 112]. However, there is an investigation reporting that the supplement of antioxidant ATRA led to reverse outcomes in IL-2 treated metastatic renal cell carcinoma patients [95]. The different outcomes of histamine and ATRA could be explained by the specificity of histamine to NOX2 which is highly expressed on myeloid cells, while ATRA calms down T cells through its antioxidative activity. Side evidence of this hypothesis is that IL-15 induces stronger activation of T cells while inducing higher ROS in T cells at the same time [135]. It is therefore suggested to be more cautious to choose appropriate ROS scavengers with more specificity to cooperate with cytokines.
ATC transfer
ATC transfer therapy refers to a strategy of infusing tumor-infiltrating lymphocytes or gene-modified T cells to combat tumor cells. ATCs hold the potential to induce complete and durable regression of metastatic human malignancies that are otherwise refractory to treatment. However, most patients with common epithelial cancers do not respond to treatment [136].
Application of ATC therapy includes three steps: separation of T cells from the host, expansion ex vivo, and infusion back to the host. A relatively low ROS level is one of the desired phenotypes of the therapeutically effective T cell [137]. The use of antioxidants during ex vivo T cell expansion has been reported to promote a more potent anti-tumor immune response in mouse models of ATC [138, 139]. Decreasing ROS through p38 inhibitor during expansion also improved the efficacy of ATCs from both mice and humans [137]. Moreover, infused T cells would encounter ROS in the tumor microenvironment, which suppresses the anti-tumor activity of T-cells, to maintain the anti-tumor activity in the oxidative tumor microenvironment. When modifying chimeric antigen receptor T cells with a co-expressing catalase, the amount of intracellular catalase is increased and the intracellular oxidative state is reduced [140]. These modulating strategies prompt that scavenging ROS of T cells plays a positive role both in the ex vivo expansion and in the in vivo activity of ATC transfer. Scavenging ROS in T cells at the in vitro expansion stage is well-described to avoid exhaustion of T cells. Inhibiting ROS in the tumor microenvironment also facilitates the anti-tumor effect of ATC therapy.
Vaccines
ROS is largely involved in the preparation and application of vaccines. Antigens are the most important components of vaccines despite the type of vaccine, and efforts have been made intensively to discover and develop specific antigens for cancer vaccines [141, 142]. Modification of existing antigen is a feasible route to obtain a stronger immune response. Oxidation of defined antigens allows protein unfolding and increases both proteolytic processing and exposes peptide epitopes that are recognized by specific T cells [143]. To stimulate the anti-tumor immune responses, Tanyi et al. [144] employed HOCl-oxidized whole tumor lysates fed to autologous DCs, followed by the therapeutic vaccination of the latter, in a cohort of ovarian cancer patients. Similarly, ROS-oxidized antigens also show higher immunogenicity. As stated above, gas plasma can generate various types of ROS, and recently, a research group has demonstrated that ovalbumin (OVA) antigen after oxidative modification by gas plasma-derived ROS led to significantly decreased tumor growth of OVA expressing melanoma compared to naive untreated OVA [78]. The researchers also proposed the concept of gas plasma technology-mediated multi-ROS-modified autologous tumor vaccine [145]. The concept “in situ vaccine” aroused from ICD and the release of tumor-specific antigens, ROS is largely involved in the formation of in situ vaccine, due to its ability to induce ICD [146].
The next step of the vaccine action is that DCs present the antigens to T cells and activate T cell responses. Adjuvants are used to stimulate a stronger immune response when administrated together with antigens. During this process, T cells and DCs elevate intracellular oxidation states upon antigen-specific interaction through the generation of ROS. An antioxidant, ebselen, inhibited DC-induced proliferation and cytokine production by T cells as well as T cell-induced cytokine production by DCs [147], implying ROS-producing adjuvants could boost the effect of the vaccine. ROS-producing adjuvants are also applied in vaccines. It has been clarified that cationic 1,2-dioleoyl-3-trimethylammonium-propane (DOTAP) liposome can stimulate DCs and induce chemokines, cytokines and co-stimulatory molecules expression via an appropriate amount of ROS generation. Interestingly, neutral 1,2-dioleoyl-sn-glycero-3-phosphocholine (DOPC) liposome does not induce ROS in DCs but even attenuate the ROS induction as well as the DCs stimulation of DOTAP [148].
The in vivo stage of the vaccine resembles the natural T cell response process, therefore, during the administration of the vaccine, the excessive ROS in the tumor microenvironment could do “bad” to the outcome of the vaccine owing to the immunosuppressive role, therefore inhibition of ROS through administration of anti-oxidants and NOX4 inhibitor strengthens the anti-tumor activity of vaccines. In summary, usage of ROS in the preparation of antigen and adjuvant is favorable, owing to the enhanced immunogenicity and DCs activation. Tumor microenvironmental ROS need to be eliminated to remove the immunosuppression.
Immune checkpoint modulators
Immune checkpoints are defined as ligand-receptor pairs that exert inhibitory or stimulatory effects on immune responses [149]. Despite novel checkpoint molecules are emerging, the approved immune checkpoint therapies are all developed based on the cytotoxic T-lymphocyte associated protein 4 (CTLA-4) and the interaction axis of programmed cell death protein-1 (PD-1) and its ligand PD-L1 [150, 151]. Several immune checkpoints-directed antibodies have increased overall survival for patients with various cancers, however, the relatively low response rate and related adverse events are still two of the concerns of the available immune checkpoint blockade.
Physical therapies like radiation, gas plasma, and PDT, and some chemotherapies can induce the generation of ROS in the tumor site and induce ICD, which permits the release of tumor-specific antigens and secretion of pro-inflammatory cytokines to promote the activation of the immune system [51, 64, 152]. A combination of ROS-induction therapies and PD-1/PD-L1 have gained augmented outcomes in several mice models of tumors [153–158]. However, disadvantages are also related to the ROS-increasing strategies. It has been observed that ROS signals weakened functional interaction between dying cancer cells and the immune system through induction of autophagy [159]. Other than the negative roles in the induction of ICD, excessive ROS increases the percentage of Treg cells in the tumor microenvironment [50, 60] and PD-L1 expression [73–75, 160]. Systemically induction of ROS also suppresses anti-tumor T cell response through the toxicity to T cells [161–163] and immune organs [82]. For example, monotherapy of paclitaxel (20 mg/kg, once a week intravenously) or antibodies against PD-L1 (200 μg/mouse, twice a week intraperitoneally) does not show any effects to the TNBC mice model, while combinatorial treatment of paclitaxel and PD-L1 blocking antibodies significantly improves the therapeutic efficacy of paclitaxel by reducing tumor burden and increasing the number of tumor-associated CTLs [53]. Therefore, ROS-inducing therapy breaks the path for immune checkpoint blockade, while the latter acts as a “patch” to restore the anti-tumor immunity upon oxidative stress [75, 160]. Decreasing ROS may also enhance the efficacy of immune checkpoint blockade through overcoming immunosuppression. ROS scavenger “sweeps” the tumor microenvironment, and may benefit for the anti-tumor immunity in some cases. NOX4 inhibitor is reported to enhance the efficacy of PD-L1 antibodies through impairing TAF and allowing T cells infiltration [44], and antioxidant ATRA enhances the efficacy of PD-1/PD-L1 blockade through functional inhibition of MDSCs [164, 165].
In summary, the regulating direction (up or down), generating site (local or systemic), administration timing (prior, meantime, or after the administration of immunotherapy), and duration of ROS (continuous or transient) should be taken into consideration to obtain optimal combination immunotherapy regimen. These factors, as the key indicators of ROS vector, outline the overall outcome from combination immunotherapy.
Conclusion
In summary, there is no doubt that the oxidative stress microenvironment at the tumor site is a devil for tumor immunity and should be eliminated to overcome the immunosuppression. However, an appropriate amount of ROS is essential in the process of T cells activation by DC cells, so indiscriminately antioxidant treatment may have adverse effects. Throughout cancer for several years, patients are inevitably faced with different treatments, ideally, these treatment works synergically to maximize the outcome. Therefore, ROS should be carefully modulated, especially when used in combination with immunotherapies. Here we would like to make some opinions for choosing proper combined therapy for T cell-based immunotherapy from the view of ROS-modulation.
The characteristics and stratification of tumors are the basis for the selection of treatments. The tumors can be defined as “cold” and “hot” according to the infiltration of T cells in the tumor microenvironment. The former refers to no or less T cells-infiltrated tumors and the latter refers to T cells-infiltrated tumors. Adaptive T transfer, vaccines and cytokines should be given priority in the “cold” tumors. In ATC therapy, both activation and expansion are completed ex vivo. Therefore, systemic antioxidant treatment has no disadvantages and can avoid T cell depletion. However, both cytokines and tumor vaccines activate T cells in vivo. In these two schemes, systemic ROS inhibition may weaken the outcomes. For the “hot” tumors, the goal of immunotherapy is to eliminate local T cell disability, and immune checkpoint inhibitors should be given priority. Removing ROS in the tumor microenvironment can relieve the immunosuppression of local T cells and revive T cell response.
The immune checkpoint inhibitors often have poor response rates due to the absence of T cells in cold tumors, and turning the “cold” tumors to “hot” is a necessary step for the immune checkpoint inhibitors treatment of cold tumors. However, in the process of turning hot, excessive, longstanding ROS should be avoided, ROS-independent ICD inducer, improving the accuracy of treatment and targeting the immunosuppressive cells through ROS inhibitors might be feasible strategies.
The most ideal scheme is to selectively clean ROS at the tumor site. In the current clinical practice and research, most ROS inhibitors are administered systemically, antioxidants will eliminate ROS indiscriminately, and NOX inhibitors show a degree of targeting through differential expression. So, the usage of antioxidants should be cautious, which has the risk of calming down the activation of DCs and T cells. Meanwhile, efforts should be made towards the targeted delivery of ROS inhibitors to the tumor microenvironment.
Abbreviations
AICD: | activation-induced cell death |
ATC: | adoptive T cell |
ATRA: | all-trans retinoic acid |
CTLs: | cytotoxic T cells |
DCs: | dendritic cells |
H2O2: | hydrogen peroxide |
HBO: | hyperbaric oxygen |
HMGB1: | high-mobility group box 1 protein |
ICD: | immunogenic cell death |
IL-10: | interleukin-10 |
LLC: | Lewis lung carcinoma |
MDSCs: | myeloid-derived suppressor cells |
NOX: | nicotinamide adenine dinucleotide phosphate oxidase |
O2•−: | superoxide radicals |
OVA: | ovalbumin |
PD-1: | programmed cell death protein 1 |
PD-L1: | programmed death ligand-1 |
PDT: | photodynamic therapy |
ROS: | reactive oxygen species |
SDT: | sonodynamic therapy |
SkQ1: | 10-(6’-plastoquinonyl) decyltriphenylphosphonium |
TAFs: | tumor-associated fibroblasts |
TAMs: | tumor-associated macrophages |
TCR: | T cell receptor |
TGF-β: | transforming growth factor beta |
TNBC: | triple-negative breast cancer |
Treg: | regulatory T |
Declarations
Author contributions
TW retrieved concerned literatures and prepared the of manuscript; HX discussed and revised the manuscript. Both of the authors edited, reviewed and approved the manuscript prior to submission.
Conflicts of interest
The authors declare that they have no conflicts of interest.
Ethical approval
Not applicable.
Consent to participate
Not applicable.
Consent to publication
Not applicable.
Availability of data and materials
Not applicable.
Funding
This work was supported by the National Key R&D Program of China (2017YFA0205504), the National Natural Science Foundation of China (Grant Nos. 81870133) and CAMS Innovation Fund for Medical Science (CIFMS2021-1-I2M-026). The funders had no role in study design, data collection and analysis, decision to publish, or preparation of the manuscript.
Copyright
© The Author(s) 2022.