Abstract
Lung tuberculosis (TB) remains a heavy burden on public health worldwide. This review discusses mainly the mechanisms of the development of pulmonary fibrosis in an experimental TB model in mice. The involvement of individual components of the extracellular matrix, the activity of matrix metalloproteinases, and the role of their tissue inhibitors in the fibrosis development. The current TB therapy activates fibrosis along with anti-mycobacterial action. The paper describes the authors’ results of experimental use of the liposome-encapsulated dextrazid (LЕDZ) combined with isoniazid (INH) which has both antifibrotic and anti-mycobacterial effects to be considered for future treatment.
Keywords
Lung fibrosis, tuberculosis, extracellular matrix, collagen, glycosaminoglycans/proteoglycans, matrix metalloproteinases/tissue inhibitors of metalloproteinases, bacillus Calmette-Guérin, liposome-encapsulated dextrazideIntroduction
Tuberculosis (TB) is a chronic infectious granulomatous disease caused by the Mycobacterium tuberculosis (Mtb) and for many years, remains one of the leading causes of death among infectious diseases worldwide [1]. About 90% of TB patients are adults, and there are more cases among men than women. TB is transmitted between people by airborne droplets and most often infects the lungs, but may disseminate to other organs, being a systemic disease characterized by a variety of clinical forms and complications.
Lung TB affects the pulmonary parenchyma, including respiratory bronchioles and alveoli [2]. The disease is characterized by the development of serious complications, such as fibrosis, cavities, bronchiectasis, and calcification [3]. The development of fibrosis is associated with a dys-regulation of the wound healing process [4, 5]. Fibrosis in TB can develop not only in untreated patients, but can also occur in those who have undergone treatment [3, 6], or during current treatment [7]. It is considered the most common type of residual lesion that occurs in the lung parenchyma [8, 9]. In patients, foci of pulmonary fibrosis are diagnosed by X-ray examination of the chest. Menon et al. [10] found that 40.4% of patients had residual lesions including fibrosis (38.7%), cavities (21.4%), bronchiectasis (4.3%), and calcification (3%). According to computed tomography, parenchymal complications included fibrosis with architectural distortions and loss of volume (90%), cavities (21%, with aspergillomas noted in 19% of these cases), and tuberculomas (54%) [11]. Respiratory tract damage was noted in the form of bronchiectasis (77%) and bronchial stenosis (4%), but no one patient had broncholithiasis.
Traditionally, lung fibrosis has been considered a result of predominantly chronic inflammation [12–14]. However, as shown in an experiment on a mice model of Mycobacterium bovis bacillus Calmette-Guérin (BCG)-induced generalized inflammation, fibrosis develops early, more precisely, 3 days after infecting, even before the development of destructive processes in the organ and chronic inflammation [15]. A month after infection, the numerical density of BCG granulomas increased by 5 times, their diameter by 6 times, the volume density of collagen fibers in lung tissue and in granuloma by 14 times and 22 times, respectively, the number of fibroblasts in the granuloma by 5 times. Such a ratio between the volume density of collagen fibers and the fibroblasts number in a granuloma allows to suppose that there are sources of fiber formation other than fibroblasts.
Apart from the increase in diameter of usual granulomas, among them there appeared new ones filled with epithelioid cells, lymphocytes, and macrophages appeared in granulomas [16]. The organism uses tumor necrosis factor (TNF) to fight Mtb infection. It has been established that the structural integrity of granulomas is maintained by TNF, and its absence leads to the destruction of granulomas with the spread of Mtb, and suppression of the protective anti-TB immune response [17, 18]. The presence of TNF and transmission of lymphotoxin α signals are necessary for the activation of immune system cells [19]. TNF and nitric oxide have been demonstrated to have a bactericidal effect in BCG-infected mice [19]. There is an opinion that TNF performs a protective role against pathogens if it is produced in the right place, at the right time, and in appropriate concentrations [20]. Mice with TNF deficiency developed reactivation of infection with a high bacterial load in the lungs, spleen, and liver [21]. At the same time, it was shown that selective neutralization of TNF with preservation of membrane TNF provides control of TB infection, retains the desired anti-inflammatory effect, but reduces the risk of infection [17].
On the other hand, excessive TNF production can cause autoimmune and inflammatory diseases, in particular rheumatoid arthritis [22, 23].
In the TB development, the leading role belongs to the immune system and the extracellular matrix (ECM) that is represented by cells and non-cellular elements such as collagens, proteoglycans (PGs)/glycosaminoglycans (GAGs), and glycoproteins.
The peculiarity of TB pulmonary fibrosis is that inside granulomas, specific formations consisted of a cluster of epitheloid cells containing bacilli of Mtb, and fibrosis around granulomas. Fibrous granulomas are associated with both good and bad outcomes of the disease. Fibrosis surrounding granulomas may be beneficial for the host, as the infected tissue is isolated from healthy parenchyma. However, fibrosis can reduce respiratory function, since the lung parenchyma is replaced by fibrotically altered tissue (scars) [24]. It is known that TB granulomatous tissues of mice are relatively aerobic [25], which allows for long-term monitoring of their development (Mtb survival in a relatively aggressive environment).
TB treatment involves a combination of several medications for many months. The standard combination includes isoniazid (INH) and rifampicin (RIF), pasiniazid and rifabutin or rifapentine, etc. Such drug regimens are a difficult task, both for patients and for the healthcare system. Some medications used in the treatment of TB can cause fibrotic complications themselves and lead to drug resistance [7]. The problem of multidrug resistance, defined as resistance to two main anti-TB drugs, INH and RIF, and broad drug resistance, when resistance develops to many second-line drugs, dictates the need to design new treatment regimens, obtain new drugs or modify existing drugs in order to increase the effectiveness of action, reduce toxicity and side effects.
In patients with TB, the diagnosis of pulmonary fibrosis is based mainly on a decrease in degree of breathing according to respiratory tests, the presence of foci of fibrosis in X-ray examination, and subjective complaints (shortness of breath, cough with and without sputum, weakness, etc.).
A detailed study of the mechanisms of pulmonary fibrosis development in patients is a huge issue for many reasons, including limited availability of human lung biopsies, limited time frames, and ethical considerations. Age and the presence of comorbidities are also important factors.
Scientific progress in the field of experimental therapy relies heavily on the interpretation of data obtained from animal simulations, which are used to identify cellular interactions and molecular pathways involved in lung tissue repair and fibrosis [26]. To a certain extent, animal studies may solve the problem [27]. Animal models will identify cellular interactions and molecular pathways involved in lung tissue repair and fibrosis [26], solving in vivo fundamental issues of TB pathogenesis and bacterial physiology [27]. Using specific models makes it possible to investigate the genesis of fibrotic complications and the mechanisms of pulmonary fibrosis development in the dynamics of the process without involving patients with actual pulmonary fibrosis.
Mouse models for the development of TB drugs have an important place since the effectiveness of modern drugs and drug combinations are predictive of their effectiveness in human studies [28]. In addition, it is possible to obtain lung samples in mouse models during any stages of BCG granulomatosis development (Table 1), which allows researchers to study various stages (phases) of the disease [29]. In addition, the feasibility of modeling the process in mice is due to the fact that when the TB process is induced in the lungs, diffuse cellular infiltration develops without structural reorganization, foci of caseous necrosis [28], and intragranulomatous necrosis in lesions [29], which altogether provide a picture of “pure” fibrosis.
Studies of the composition of granulomas and interstitial lung tissue in the BCG-induced granulomatous model
Parameter | Reference | |||||
---|---|---|---|---|---|---|
[15] | [16] | [36] | [73] | [77] | [95] | |
Time after BCG infection (days) | 3, 30 | 14–28 | 3–180 | 3–180 | 30–180 | 7–28 |
Lung granulomas | ||||||
Numerical density | √ | √ | √ | |||
Diameter | √ | √ | √ | |||
Сollagen fibers | √ | √ | √ | |||
TNF-α | √ | √ | ||||
Caspase 3 | √ | |||||
TRAIL | √ | |||||
Macrophages | √ | √ | √ | |||
Fibroblasts | √ | √ | √ | |||
Epithelioid cell | √ | √ | ||||
Lymphocyte | √ | √ | ||||
Lung interstitium | ||||||
Collagen fibers | √ | √ | √ | |||
sGAG | √ | |||||
Protein | √ | |||||
Uronic acids | √ | |||||
Galactose | √ | |||||
Hydroxyproline | √ | √ | √ | |||
TNF-α | √ | |||||
TGF-β | √ | |||||
Macrophages | √ | |||||
Fibroblasts | √ |
Blank cells represent no description. TRAIL: TNF-related apoptosis-inducing ligand; sGAG: sulfated GAG; TGF-β: transforming growth factor-β; √: included in the study
The issue of the timing of the occurrence of fibrous granulomas remains relevant [6]. The study of the complex architecture and regulation of the process of lung fibrosis is the key to determining the optimal methods of TB treatment [24]. It can be noted that the modeling of the TB process makes it possible to clarify the timing and speed of fibrosis development, identify the main players and regulators of this process, as well as to assess the effect of anti-TB drugs on the severity of fibrosis. Several models of lung fibrosis have been developed and are being used, including the bleomycin model. However, BCG-induced inflammation (Table 1) caused by Mtb in the BCG vaccine or live strains of virulent forms of Mtb is more often used to reproduce TB fibrosis. In this paper, the main attention is paid to the consideration of the mechanisms of pulmonary fibrosis reproduced on a model of BCG-induced granulomatosis in mice. Discussed are the cellular composition of granulomas, the components of lung ECM, the activity of matrix metalloproteinases (MMPs), and the content of tissue inhibitors of metalloproteinases (TIMPs) after infection of animals for 6 months as well as the results of the use of a drug composition liposome-encapsulated dextrazide (LEDZ) created on the basis of INH with oxidized dextran (OD) in liposomal form as an anti-TB drug candidate for reducing severity of pulmonary fibrosis.
Fibrosis in pulmonary TB
The development of pulmonary fibrosis is always preceded by damage to the alveolar epithelium. In TB, Mtb acts as a damaging factor. In all injuries, fibroblasts are the main type of cells responsible for wound healing and fibrosis development [4, 30–34]. However, other cells may be involved in this process. Epithelial cells take part in immunoregulatory processes, and secrete pro- and anti-inflammatory cytokines [35], which can directly or indirectly affect the metabolism of the ECM of the lungs. In addition, they produce lipocalin-2, which binds mycobactin and mycobacterial proteins, and thereby disrupt the metabolism of Mtb. The key influence of the endothelium on Mtb infection is the expression of vascular cell adhesion molecule 1 (VCAM-1) adhesion molecules.
Generalized TB inflammation is characterized by the formation of granulomas in different organs from phagocytic resident and recruited cells, the ratio of which reflects the “age” of the granuloma. On the BCG-granulomatosis model, the number of macrophages in granulomas decreased by 2.3 times: It was maximal at 3 days (56%) and decreased by 180 days (25%) after infecting [36]. At the same time, the number of fibroblasts changed in a reverse order: In granulomas, it increased more than 15 times (from 1.2% on 3 days to 19.8% on 180 days), and it increased 4.5 times in lung interstitial tissue (outside granulomas) from a numerated density of 1.2 on 3 days to 5.6 on 180 days. The noted decrease in the number of macrophages in granulomas may be associated with the macrophage-to-myofibroblast transformation (MMT) [7] and indicate the stabilization of the inflammation process and the beginning of its chronification, as well as an increase in the number of fibroblasts in granulomas, especially from the 90 days of inflammation.
It is known that in the acute phase of infection, TNF from myeloid cells (macrophages/neutrophils) is provided for protection, whereas TNF from T cells is needed to maintain protection in the chronic stage [37]. It is possible that the redistribution of cells in granulomas is regulated by TNF from different sources.
A decrease in the number of macrophages in the dynamics of granuloma development and an increase in peripheral granuloma fibrosis occurs both due to fibroblasts/myofibroblasts granuloma, as well as due to MMT [7]. This mechanism, according to the authors, underlies fibrosis associated with granuloma. To some extent, the mechanism of MMT can explain the data on a significant increase in the volume density of collagen fibers in the granuloma within a month after infecting (22 times) relative to the number of fibroblasts in the granuloma, which increased only 5 times [15]. Granulomas recruit inflammatory dendritic cells that can regulate local T-cell responses and may play a direct role in the spread of granulomatous inflammation [38].
While granuloma fibrosis provides a certain degree of encapsulation of the pathogenic locus, interstitial pulmonary fibrosis, as a rule, only aggravates the disease due to gas exchange disorders, since granulomas and collagen deposits in the interstitium reduce the respiratory surface of the lungs. Fibrotic complications in granulomatosis of various etiologies are associated with chronic inflammation associated with the “maturation” of granulomas, the appearance of destruction loci in the lung parenchyma, and the development of defective reparative processes. In this regard, the most frequent complication of TB inflammation is respiratory failure, which requires appropriate treatment and prevention of fibrosis of the organ, in addition to suppressing the infectious onset—Mtb.
The main components for fibrosis of the lungs in TB, as in many other diseases, are the main components of ECM, as well as compound regulating their metabolism (the MMPs and TIMPs family).
ECM of the lungs
The ECM in the mammalian body is a complex and highly labile system that responds to effects of endogenous and exogenous factors by structural rearrangements of its main components. The main components of ECM include collagens, PGs, and glycoproteins.
Collagens
In turn, collagens are divided into several classes. Among the known XXIX types of collagen [39], most of which belong to non-fibrillar collagens, a smaller part constitutes fibrillar collagens [40, 41]. The latter group (type I, II, III, V, and XI) performs the formative and biomechanical function of ECM and prevents tissue compression. In the lungs, type I and type III collagens are involved in the mechanics of respiratory movements [42] and, along with surfactant, they maintain alveoli in a straightened state. Type IV collagen, having a lamellar structure with fenestrae, is part of the basement membrane of endothelial, epithelial, and other cells. Lung ECM macromolecules (collagen and elastin) are the main factors determining the mechanical properties of lung tissue. Therefore, changes in the composition of these components and their structural organization affect the physiological characteristics of the lungs [43–45]. There are relationships between tissue composition, microstructure, and macrophysiology, showing that the physiological behavior of the lungs reflects both the mechanical properties of individual tissue components and their complex structural organization [46–48], the imbalance of which can lead to impaired ventilation and perfusion, the development of tissue hypoxia and respiratory failure.
Fibrosis is characterized by excessive production of ECM components, primarily collagen, by fibroblasts and other types of cells [49, 50], such as macrophages, endothelial cells, alveolar, and bronchial epithelial cells that can produce components of ECM after lung damage [12].
ECM, present in all organs and tissues, is a complex structural and functional formation [51], changing the process of growth and development of the body [52, 53]. At the same time, the maintenance of homeostasis of the ECM ensures the implementation of its main functions, in particular, the provision of structural, trophic, signaling, intercellular interactions, etc.
In pathological conditions, the ratio of macromolecular structural components of ECM [52, 54], signaling molecules [55], and the activity of enzymes involved in anabolic and catabolic reactions [56, 57] change (Figure 1A and B).
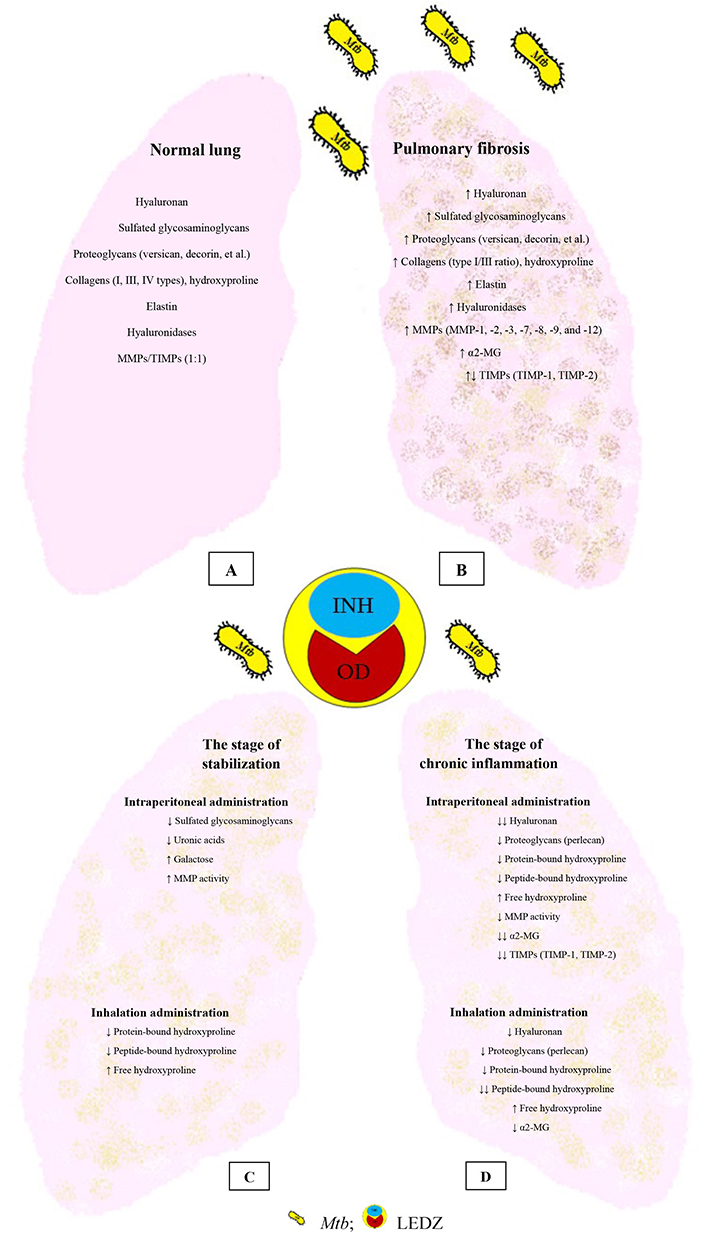
The main components of the lung ECM are normal, with TB and the introduction of LEDZ. (A) Normal lung; (B) pulmonary fibrosis; (C) the effect of LEDZ administration at the stabilization stage; (D) the effect of LEDZ administration at the stage of chronic inflammation. α2-MG: α2-macroglobulin. ↑: increase in the indicator; ↓: decrease in the indicator; ↓↓: a greater reduction than ↓
The appearance of type I collagen and myofibroblasts around granulomas indicated the initial stage of the forming of a fibrous membrane in this place. In addition, in lung TB, aminoterminal propeptides of procollagen types I and III were found in histological samples accumulated to a greater extent around granulomas than inside them [58], which indicates activation of collagen synthesis of types I and III more in the interstitial than inside granulomas. However, evidence exists that collagen fibers of type I are 12 times more often detected inside granulomas than outside, collagen fibers of type III and elastic fibers 5.4 times and 5.6 times, respectively [59]. It seems likely that such a difference in collagen content is determined by the age of granulomas, fibrosis phase, and duration of the disease.
The development of fibrosis in the lungs occurs at a variable rate. This is evidenced by the results of studying the content of hydroxyproline in the lungs of BCG-infected mice for 180 days (6 months) [36]. On the 3rd day after infecting, the content of hydroxyproline, a marker of collagens has already increased by almost 2 times relative to the control, on the 30th day it increased 1.5 times compared to the 3rd day, on the 60th and 90th days there was a downward trend, but on the 180th day the maximum content of hydroxyproline was twice as much as compared with the data of 3 days and 1.3 times higher compared to 30 days.
The results of the immunohistochemical study of the content of fibrous connective tissue in the lungs correspond to the data obtained for the content of hydroxyproline in different stages of BCG-induced inflammation. The finding confirms that the fibrosis development in the lungs begins as early as 3 to 30 days from the beginning of infection (the stage of acute inflammation). A slight decrease in these indicators by 60 and 90 days indicates the stabilization of fibrosis (stabilization stage), while the subsequent increase in the chronization of the process by 180 days can be considered as a stage of chronic inflammation. Thus, the dynamics of the hydroxyproline content in the lungs were characterized by stage. It increased by 30 days (the stage of acute inflammation), decreased by 60 and 90 days (the stage of stabilization), then increased again to a maximum value by 180 days (the stage of chronic inflammation).
The change in the lung content of hydroxyproline may be associated either with an increase in collagen synthesis (due to an increase in the number of fibroblasts without increasing their fibroplastic activity; an increase in their fibroplastic activity without increasing the number) or the intensity of degradation of synthesized collagen. The severity of pulmonary fibrosis in chronic granulomatous inflammation is primarily determined by the number, size of granulomas, and the degree of their “maturity”, which determines its cellular composition [60]. The dynamics of the hydroxyproline content in the interstitial of the lungs, apparently, is due to both a progressive increase in the numerical density of granulomas from 3 to 180 days, and an increase in their size [36].
PGs and GAGs
PGs and GAGs, as well as collagens, are involved in TB granulomogenesis and fibrosis. All GAGs, except hyaluronan, contain sulfate groups and are called sGAG. They constitute disaccharide chains of alternating monomers (N-acetylated hexosamine—glucosamine or galactosamine) and hexuronic acids (D-glucuronic acid or L-iduronic acid). PGs consist of a basic protein and covalently attached negatively charged sGAG.
According to aggregation PGs in the lungs are divided into three classes [61]. They constitute large chondroitin sulfate PG (versican), small leucine-rich PGs (decorin, biglycan), and heparan sulfate PGs (collagen type XVIII, perlecan, and agrin). Syndecans of types 1–4 are singled out separately [62].
It is known that in lung TB the connective tissue surrounding granulomas contains versican, large chondroitin sulfate PG, and myofibroblasts. In myofibroblasts, type I procollagen was intracellularly stained [63]. Versican (chondroitin/dermatan sulfate PG) is produced by smooth muscle cells and fibroblasts [64]. An interstitial decorin, containing one chain of chondroitin sulfate/dermatan sulfate, was localized in epithelioid cells of granuloma and some myofibroblasts [63]. As a rule, it is closely related to collagen fibrils [65, 66]. According to the authors [63], collagen synthesis is preceded by producing a preliminary matrix rich in versican, i.e., myofibroblasts at first synthesize versican, and after its content reaches sufficient value in the surrounding ECM, they begin to synthesize collagen.
PGs and GAGs are present in ECM and plasmalemma of almost all animal cells, they exist among host macromolecules primarily encountering infectious agents [67]. Hyaluronan, unsulfated GAG, is detected diffusely throughout the lung ECM and performs a structural role. Besides, it can affect many cellular functions and processes, including inflammation [68]. The involvement of hyaluronan in macrophage aggregation during the formation of the primary nucleus of TB granuloma via CD44 receptors is also discussed [49].
GAGs are the “first line” contact with the pathogen and host cells involved in the process of adhesion and invasion [69], modulating the process of inflammation [70]. There are 4 classes of GAGs in the lungs [71], in particular, hyaluronan (14%, 1st class), chondroitin sulfate and dermatan sulfate (31%, 2nd class), heparan sulfate (40–60%) and heparin (5%, 3rd class), keratan sulfate/keratan (4th class).
In the lungs of intact mice, 70–90% of sGAGs are heparan sulfate and chondroitin sulfate/dermatan sulfate [70]. Their content and qualitative composition change with inflammation (Figure 1B). In particular, an increase in sGAG and PGs was shown in patients with pulmonary TB [63]. It is noteworthy that versican, sGAG, and hyaluronan were located in the lung ECM along the edge of developing granulomas, whereas decorin is situated intracellularly in epithelioid cells.
Most of the hyaluronan exists in the ECM in the free state and only 1% binds with hyaladherins [40]. During infection and formation of the “primary nucleus” of granuloma, an important role is assigned to hyaluronan, which participates in the recruitment of leukocytes through its interaction with CD44 [72] and provides aggregation of macrophages in granulomas [49]. Additionally, hyaluronan is one of the main GAGs of ECM, which is regulated by TGF-β, and whose role in fibrosis has been proven [54].
As it was noted above, PGs consist of a basic protein, uronic acids, and hexosamines. It is of interest to study the structure of PGs in TB, as this will help to assess the contribution of individual PGs components in the development of fibrosis.
The study of the structure of PGs in the lungs demonstrated their maximum content on 30th days after BCG-infecting in mice [73]. The sGAG content for 30 days was higher relative to the control. After 30 days, it decreased by 1.9 times on the 60th day, by 3 times on the 90th day, and 2 times lower on the 180th day compared to 30th day. The gradual increase in sGAG by 30 days coincides with the stage of active formation of granulomas, a characteristic manifestation of the TB process. The numerical density of granulomas increased 2.2 times for 30 days [36].
The content of uronic acids, galactose, and protein in PGs in the lungs increased to maximum values on day 30 and then gradually decreased. The noted maximum sGAG content in the lungs of mice on day 30 may reflect an increase in PGs around (versican) and inside (decorin) granulomas.
The development of a pathological process in a certain target organ may affect the content of GAGs in the blood serum. The content of total GAGs in the serum of BCG-infected mice at the above specified observation stages differed from the dynamics of GAGs in the lungs [73]. The total GAG content in infected animals was higher than in the control mice. Their maximum level was observed for 90 days, the minimum for 60 days. It is known that the content of GAGs in blood serum characterizes the intensity of PGs metabolism in the body [74], and the level of hyaluronan circulating in the blood is associated with the intensity of its intake from peripheral tissues through the lymph [75].
Differences in the content of total GAG and sGAG at different infection stages suggest that the synthesis of sGAG was activated in the lungs of BCG-infected mice for 30 days, since no increase of GAGs in the serum during this stage was found. An increase of serum total GAG by 90 days against the decrease in sGAG in the lungs may be due to a shift in the GAGs composition in favor of hyaluronan. The latter is probably formed as a result of degradation of GAGs under both host and bacterial hyaluronidases activity.
It is known that heparan sulfate PG the CD44 receptor, actively participates in the catabolism of hyaluronan, creating favorable conditions for the action of hyaluronidases and the formation of low-molecular fragments of hyaluronan [40]. These fragments are involved in various signaling events, including cell proliferation, migration, differentiation, and induction of proinflammatory cytokines and chemokines [68, 72].
Changes in the metabolism of PGs/GAGs in the lungs associated with granulomogenesis are not always reflected in the blood serum. This concerns the ability of PGs and hyaluronan to facilitate the adhesion of leukocytes and their migration, to interact between PGs and MMP, while reflecting the direct regulation of MMPs activity [70]. An increase of total GAGs level in blood serum by 90 days with a simultaneous decrease in sGAG in the lungs during this stage indicates the possible involvement of low molecular weight hyaluronan in the development of inflammation and increased fibrosis of the lungs by 180 days.
During the breakdown of macromolecular aggregates consisting of hyaluronan and PGs, the released hyaluronan can be exposed to mycobacterial hyaluronidases with the formation of low molecular weight hyaluronan, which has a pro-inflammatory effect. However, another mechanism of GAGs increase in TB inflammation is not excluded, associated with increased expression of hyaluronan synthase and accumulation of hyaluronan on the surface of epithelial cells of the respiratory tract, alveoli, and around granulomas in the lungs of infected mice [76]. An interesting fact is that Mtb uses the host’s ECM hyaluronate for their growth. Degradation of PGs, including versican, the content of which is increased in lung TB [63] may be a source of increased sGAG content.
In chronic BCG-induced inflammation, the expression of TNF-α by activated macrophages was noticeable from day 30, and it was 4.5 times more intense in the interstitial lung than in granulomas [36]. At 180 days of the infectious process, the expression of TNF-α was almost 2 times higher compared to that at 30 days and coincided with the maximum content of hydroxyproline in the lungs. It appears likely that the increase in lung fibrosis by the end of the experiment, as it is evident from the volume density of fibrous tissue and the content of hydroxyproline in the lungs, was induced by a high content of low-molecular-weight hyaluronan fragments with a pro-inflammatory effect in the blood serum for 90 days.
In BCG granulomas (30–90 days), the total number of cells, altered by apoptosis, increased by more than 20% [77]. It has been established that two apoptotic mechanisms are involved in this process: The receptor-mediated mechanism of apoptosis (TRAIL) is more pronounced and associated with cells expressing proinflammatory cytokine (TNF-α) than mitochondrial—mediated caspase 3. However, this does not inhibit the persistence of the pathogen in macrophages of granulomas but contributes to the formation of pronounced fibrosis in granulomas and interstitial lung.
Thus, in the mouse model of chronic BCG-induced inflammation, it was shown that the structural components of PGs in the lungs were maximally elevated for 30 days, they decreased in subsequent stages, which indicates the stage of PGs metabolism in the dynamics of fibrosis development.
The study of sGAG in the dynamics of chronic TB inflammation allowed getting an idea of their contribution to their structural diversity of developing destructive and especially fibroplastic processes, one of the main complications of TB, which are fully manifested 6 months after infecting.
It is known that bacteria, including Mtb, use disaccharide chains of PGs/GAGs as co-receptors for their adhesion and subsequent invasion into the host cells [69, 78]. It is believed that Mtb adhesion to alveolar macrophages and their phagocytosis can be facilitated by surfactants of types A and D present in the lung alveoli [79]. Mtb adhesion is a mandatory stage of interaction for the subsequent formation of granulomas. Their significance is different: For the host organism, granuloma restricts infection, for Mtb—It protects from immune attacks and maintains viability [80]. In 10% of latently infected individuals, bacilli can reactivate with a detailed clinical picture of the disease. The prognosis of the disease is largely determined by the host’s ability to eliminate Mtb.
Taking into account the “mandatory” stage of PGs/GAGs interaction with Mtb during the formation of granulomas, the expediency of developing drugs that suppress an attachment of the pathogen to the disaccharide chains of GAGs is discussed [69]. Inhibition of adhesion could prevent the invasion of Mtb into host cells, the formation of granulomas, and the long-term persistence of Mtb in them.
The quantitative assessment of PGs components in the lungs in the mice model of chronic BCG-granulomatous inflammation showed the lability of their structure at different stages of the process, reflecting the replacement of one GAGs by another. A gradual increase in sGAG, UAs, protein, and galactose during the acute stage of inflammation with a maximum on the 30th day may characterize the intensity of the response of ECM to Mtb infection with the formation of granulomas. However, the dynamics of the content of PG components differ from the dynamics of the content of hydroxyproline.
MMPs/TIMPs
Homeostasis in the ECM and the exchange of its components is regulated by a complex system of enzymes. The main enzymes that destroy ECM molecules are MMPs, a disintegrin-like and metalloproteinase domain with thrombospondin type 1 repeat (ADAMTS), and their activity is controlled by endogenous inhibitors, TIMPs [81–87]. The MMPs synthesis is regulated at the transcription level, and their proteolytic activity is controlled by the activation of proenzymes, as well as the inhibition of active enzymes by endogenous inhibitors—TIMPs and α2-MG, which play an important role in fibrosis processes [88, 89]. It is usually assumed that elevated levels of TIMPs lead to the accumulation of ECM, causing fibrosis, while their decrease leads to enhanced matrix proteolysis [90].
The MMPs family of zinc-dependent endoproteases consists of 28 representatives, whereas TIMPs are a family of four endogenous proteins [91]. Depending on the substrate specificity and domain organization, MMPs are subdivided into collagenases, gelatinases, stromelysins, matrilysins, membrane-type MMPs, and other MMPs [82, 92, 93].
The expression of MMPs and TIMPs by cells is regulated by many cytokines [in particular, interleukin-1 (IL-1)], growth factors, and hormones, some of which are specific to the cell type, while others are ubiquitous (for example, TGF-β) [94]. High levels of TGF-β1 were detected in tissue fibrosis [4, 95, 96] and fibrous granulomas [6].
The profibrotic properties of TGF-β1 are associated with its participation in stimulating the proliferation, differentiation of fibroblasts, and their transformation into myofibroblasts [97, 98]. It is known that TGF-β induces connective tissue growth factor (CTGF) in fibroblasts through various signaling pathways [99, 100]. Only when interacting with TGF-β, CTGF over expression and pronounced fibrosis were observed in vivo [101, 102]. The effect of another cytokine IL-10 consisted in reducing fibrosis and inhibiting the synthesis of TGF-β1 during pulmonary fibrosis [103–106].
MMPs and TIMPs are considered as potential regulators of TB infection [107]. It was shown that the levels of MMP-1, MMP-8, MMP-9, and MMP-12 in blood plasma were significantly increased in patients with pulmonary TB compared to individuals with extra-pulmonary TB, latent TB, and healthy control. On the contrary, the level of MMP-7 was significantly reduced in patients with pulmonary TB compared to those with extra-pulmonary TB. Similarly, the levels of MMP-1, MMP-7, and MMP-13 were significantly increased in patients with extra-pulmonary TB compared to those with latent TB and healthy control. However, the level of TIMP-2 was significantly reduced, and TIMP-3 was significantly increased in people with extra-pulmonary TB compared to latent TB and healthy control.
When infected with Mtb, MMPs regulate cell migration, reconstruct lung tissue, and initiate granuloma formation [108]. A violation of the regulation of MMPs activity leads to the progression of various pathologies, which can be grouped into: 1) tissue destruction, 2) fibrosis, and 3) matrix weakening [86]. Both “classical immune cells” of myeloid and lymphoid origin and “non-classical immune cells” are involved in all these processes [35]. Type II alveolar pneumocytes infected with Mtb secrete MMPs, which destroy collagens, and contribute to the formation of cavities and the spread of bacilli. The destruction of lung tissue with the formation of caseous necrosis and caverns [109] is also facilitated by the activation of collagenase—MMP-1 associated with the accumulation of hypoxia-inducible factor-1α [83].
Numerous ex vivo and in vitro studies demonstrate that Mtb induces the secretion of MMP-1 by host cells in an inactive form—pro-MMP-1, requiring proteolytic activation [110]. In addition, Mtb secretes serine proteases [111, 112], which indicates a potential proteolytic cascade in which Mtb both directly induces and activates MMP-1 in its microenvironment, contributing to the destruction of the matrix.
The participation of MMPs in the pathogenesis of TB is evidenced by the results of studies of other MMPs. There was a significant increase in the level of MMP-1 by 15 times, MMP-3 by 3.6 times, but a decrease in TIMP-1, TIMP-2 in sputum and bronchoalveolar lavage fluid in patients with pulmonary TB relative to control [113]. However, MMP-8 was not detected, and the content of MMP-2, MMP-7, and MMP-9 did not differ from the control. At the same time, it was shown that the level of circulating MMP-9 was increased in patients with TB relative to the healthy individuals and correlated with the severity of TB [114]. The content of MMP-2 did not differ from the level in the control. In addition, in transgenic mice that express human MMP-1 in the lungs, Mtb infection increased the expression of MMP-1, which led to alveolar destruction in lung granulomas and significantly greater collagen breakdown compared to wild mice.
In patients with infiltrative pulmonary TB with pronounced fibrous changes (70% of patients with multidrug resistance) after the intensive phase of chemotherapy, a more than 8 times decrease in the content of MMP-9 was noted [115]. However, no changes in other enzymes, pro-MMP-1, MMP-8, TIMP-1, and α2-MG were detected. With positive clinical dynamics and complete closure of destruction, the content of MMP-9 decreased by 4.5 times, while in maintaining destruction and decay cavities it fell only by 1.2 times.
Another study showed that in patients at the end of TB treatment, the concentration of MMPs remained elevated due to the influence of pro-inflammatory chemokines [116]. The persistent increase in TIMP-1 and TIMP-2, noted after 6 months of treatment, is associated with ongoing tissue remodeling and possible fibrosis.
An imbalance in the MMPs/TIMPs system leads to degradation of the connective tissue ECM and pathological remodeling, forming a morphological basis for violations of external respiration. This is evidenced by the correlations found in pulmonary TB patients between the concentrations of MMP-9 and MMP-8 in the blood with the extent of lesion of the pulmonary parenchyma when comparing tuberculomas and fibrous-cavernous TB, MMP-9, and TIMP-1—with changes in pulmonary volumes, MMP-8—with functional disorders of gas exchange. However, changes in MMP-1, MMP-3, and α2-MG did not correlate with the volume of lesion of the pulmonary parenchyma and with a decrease in lung function [47].
When BCG was administered to CBA mice 1.5 months after being infected, an increase in the total activity of MMP-2 and MMP-7 in the blood serum was observed [117]. According to the authors [117], such an increase, on the one hand, can ensure the migration of macrophages to the focus of inflammation and lead to the formation of granulomas, and on the other hand, indicate the development of destructive processes in the ECM.
The immunohistochemically measured MMP-9 and the number of myofibroblasts are detected 9 and 12 times more often, respectively, inside granulomas than outside them [59].
There are observations that over expression of TIMP-2 increased the activity of autophagy and decreased the levels of IL-6 and TNF-α in Mtb-infected A549 cells [118]. An increase in the expression of MMP-1 and a decrease in TIMP-3 in New Zealand rabbits is associated with the progression of TB by the formation of cavities in the lungs with a high bacterial load [119].
Incubation of peritoneal mouse macrophages with viable BCG and studies in vivo with infection of BALB/c BCG mice showed increased secretion of MMP-9 and activity of the enzyme [120]. Exogenous addition of TNF-α or IL-18 induced expression of macrophages by MMPs, whereas immunoregulatory cytokines, interferon-γ, IL-4, and IL-10 all suppressed BCG-induced MMP production.
Intravenous infecting of C57BL/6 mice with virulent Mtb Erdman strain leads to differential expression of MMPs in the lungs [121]. The activity of MMP-9 increased by 1 week after infection, while the activity of MMP-2 after 2 weeks. Reverse transcription polymerase chain reaction (RT-PCR) analysis for the expression of gelatinase genes and their corresponding inhibitors showed a slight increase in MMP-9 by 1 week, no changes were observed in TIMP-1 and MMP-2, as well as a significant decrease in TIMP-2 by 4 weeks. According to the authors [121], glycolipid lipoarabinomannan and other Mtb components induce the expression of MMP-9. In the mouse model of BCG-induced inflammation, 6 months after infection, the total activity of MMPs increased by 9 times, hyaluronidase by 4 times, α2-MG by 10 times, TIMP-1 by 11 times, and TIMP-2 by 13 times in the lungs [122, 123], that indicates high both protease and antiprotease activity of enzymes.
The participation of the MMPs/TIMPs system in the mechanisms of fibrosis regulation is multifaceted. It is known that collagenases (MMP-1, MMP-8, and MMP-13) reduce fibrosis by degradation of collagens, MMP-2—by reducing the expression of type I collagen, MMP-12 indirectly stimulates fibrosis by suppressing MMP-13 and activating fibroblasts, MMP-7 indirectly mediates fibrosis by increasing the influx and activation of neutrophils that stimulate tissue destruction, epithelial damage and increase fibrosis [57].
An evaluation of the activity of the MMPs/TIMPs system in the lungs in an animal model, apparently, characterizes the direct responses of enzymes to infection and treatment whereas clinical data obtained by measuring biochemical parameters in serum, plasma, sputum, and bronchoalveolar lavage fluid provide oblique results. However, in both cases, the results can vary depending on the stage of the inflammatory process, on scheme and time of initiation of treatment, comorbidity, and the development of complications.
LEDZ
For the treatment of TB patients, various regimens have been developed, depending on the severity and duration of the process. Several drugs have been used for a long time that are not devoid of toxic properties. In some patients, such therapy causes multiple and broad drug resistance, which forces to change treatment regimens, medications, and methods of their administration. Often, the weak effectiveness of medicines is due to the untimely (late) start of treatment.
In this section of the review, the results of the use of a special drug composition in mice with BCG-induced inflammation will be discussed. It is the first-line anti-TBs drug INH combined with OD [molecular mass (MM) 40 kDa], notably dextrazide conjugate enclosed in liposomes (0.20–0.25 microns), which is a liposomal form of dextrazide (LEDZ). Dextrazide is known to have low toxicity, prolonged action, selectivity of accumulation in the vacuolar system of macrophages, and suppresses Mtb growth [60]. The study was performed on a BCG-induced granulomatosis model, since there is data that the effectiveness of modern drugs and their combinations on a mouse model generally corresponds to their effectiveness in human studies [28].
The evidence of inhibition of pulmonary fibrosis in TB by OD is based on the results of reducing the number of adhesions in the abdominal cavity and the volume density of collagen in them [124], as well as reducing the content of protein-bound hydroxyproline and total GAG in blood serum in a model of adhesive disease in rats [125]. The data on the effectiveness of LEDZ in mice are presented below, depending on the method of administration and the stage of the TB process.
Introduction of LEDZ at the stage of stabilization of BCG-induced granulomatosis
Intraperitoneal administration of LEDZ at the stage of stabilization of BCG granulomatosis in mice (3 months after infection with BCG vaccine, LEDZ administration for 2 months), when fibrotic processes in the lungs are slowed down [36, 73], was accompanied by significant changes in the structure of pulmonary PGs, a decrease in the content of sGAG, uronic acids, and an increase in galactose (Figure 1C) [126]. This, apparently, indicates in favor of an increase in the content of keratan sulfate PG, which in the lungs of intact mice is no more than 10% [70]. The introduction of LEDZ at this stage did not affect the content of the hydroxyproline and its fractions. At the same time, the activity of MMPs increased, but not the content of TIMP-1 and TIMP-2. This ratio may be associated with the activation of MMP performs and increased expression by MMP-1 and MMP-9 macrophages, since earlier in an in vitro experiment such activation of enzymes was observed when LEDZ was introduced into a culture medium [127].
LEDZ inhalations performed during the stabilization stage of BCG granulomatosis proved to be more effective in terms of reducing the severity of fibrosis in the lungs [128]. The antifibrotic effect during this stage was reproduced by an increase in degradation (i.e., through the increase in free hydroxyproline) and a decrease in collagen synthesis (via a decrease in the content of total hydroxyproline, peptide-bound hydroxyproline, and protein-bound hydroxyproline) (Figure 1C). The decrease in hydroxyproline fractions during inhalation was due to the direct LEDZ entry into the respiratory tract and alveoli followed by its capture by macrophages.
In another mouse model, intraperitoneal administration of LEDZ (4 months after infection with BCG vaccine, LEDZ administration for 2 months) suppressed the expression of MMP-9 mRNA and type III collagen α1 chain in the lungs, which indicates inhibition of fibroblast differentiation into myofibroblasts [129]. Inhalations of LEDZ in this experiment had no effect on the studied indicators.
Introduction of LEDZ at the stage of chronic BCG-induced granulomatosis
Under the intraperitoneal LEDZ administration in the stage of chronic BCG granulomatosis (6 months after infection with BCG vaccine, LEDZ administration for 3 months), the lung contents of hyaluronan, perlecan, peptide-bound hydroxyproline, and protein-bound hydroxyproline decreased, but the content of free hydroxyproline increased (Figure 1D), indicating increased degradation of collagens and suppression of their synthesis [123, 130]. The introduction of LEDZ at this stage of BCG granulomatosis led to a decrease in the activity of MMPs and specific and non-specific inhibitors (TIMP-1, TIMP-2, and α2-MG), which also implies a decrease in the activity of destructive-inflammatory potential [122]. The combined decrease in MMPs and α2-MG activity, level in TIMP-1 and TIMP-2 indicates the suppression of protease, antiprotease activity, and inflammatory response due to the influence of LEDZ.
After inhalation of LEDZ, the content of hyaluronan, perlecan, protein-bound hydroxyproline, peptide-bound hydroxyproline, as well as total hydroxyproline and an increase in free hydroxyproline in the lungs were also reduced (Figure 1D) [123, 131]. However, the activity of MMPs did not differ from the BCG group, while their inhibitors decreased, TIMP-1, TIMP-2, and α2-MG, but remained elevated compared with intraperitoneal administration of LEDZ.
Thus, this section shows the effects of LEDZ on the components of ECM and enzymes that control the exchange of PGs and collagen. The effects of LEDZ depend on the stage of infection and the route of administration. It should be noted that when LEDZ is administered, fibrolysis is activated in both routes of administration. This antifibrotic effect is more pronounced when the composition was administered in the stage of chronic BCG granulomatosis than at the stage of stabilization.
It can be assumed that the components of ECM and their local regulators involved in TB granulogenesis can serve as a target for the development of new approaches to chemotherapy that will improve the prognosis of the disease and reduce the risk of complications.
Conclusions
According to World Health Organization (WHO), there have been some successes in TB therapy, however, for many years TB remains one of the leading causes of death among infectious diseases worldwide. Among the causes for this situation is inadequate knowledge concerning the pathogenesis of the disease and the mechanisms of complications, and insufficient effectiveness of available medicines, which, as a rule, should be taken for a long time. In addition, patients’ adherence to therapy is not always existed due to the duration of treatment and the development of such side effects of anti-TB drugs as interstitial fibrosis, general toxicity, hepatotoxicity, etc.
Pulmonary fibrosis in TB is the most common type of lesion that occurs in the lung parenchyma. Contrary to previous opinions about fibrosis as a result of chronic inflammation, it can develop early in the stage of acute inflammation. Fibrosis is found in untreated people, during treatment, as well as after treatment. It is detected both around the granulomas and inside.
A detailed study of the mechanisms of pulmonary fibrosis development in patients is a huge problem for many reasons. First of all, this is the limited availability of human lung biopsy material (pathology samples), as well as time frames, ethical considerations, the age of patients, and the presence of comorbid diseases. To a certain extent, the problem can be solved on experiment animals. The use of a mouse model of TB process in its dynamics allowed us to obtain a picture of “pure” fibrosis without foci of caseous necrosis and cavities.
The study of the main components of the ECM showed that their changes are characterized by dynamism and the forming of associations between the structural units of PGs and hydroxyproline at different times of the TB process. The exploration of the dynamics of BCG granulomatosis in mice for 6 months revealed three stages: 1) acute inflammation, 2) stabilization of granulomatosis, and 3) chronic inflammation.
The effectiveness of the composition consisting of dextrazide, an INH conjugate with OD enclosed in liposomes—LEDZ, depended on the stage of granulomatosis it was administered. Such a difference in the effectiveness of LEDZ is explained by the selectivity of the effect of the composition on various links in the pathogenesis of pulmonary fibrosis. In particular, when LEDZ is introduced at the stabilization stage, the composition has a greater effect on the exchange and structure of PGs, than introduced at the stage of chronic inflammation. In this case, its effect extends to collagen exchange and the system of local regulation of ECM metabolism.
In addition, the BCG-induced inflammation model shows that the therapeutic effect of LEDZ depends on the specific route of administration. Its antifibrotic and anti-inflammatory effects were more significant under LEDZ inhalations. These data once again indicate the need and importance of following an individual approach in the treatment of TB.
The most recent, studies performed on various animal models of TB suggest using MMPs inhibitors along with first-line anti-TB drugs, i.e., INH and RIF, which significantly reduced the survival of Mtb in the lungs, prevented maturation, reduced the number of granulomas and matrix degradation. The LEDZ used in the work convincingly reduces the activity of MMPs, hyaluronidase, α2-MG and decreases the content of profibrotic fractions of hydroxyproline in the lungs of infected mice. These effects were obtained when LEDZ was administered for 3 months, which is significantly shorter than the recommended treatment time (6 months or more).
Abbreviations
BCG: |
Mycobacterium bovis bacillus Calmette-Guérin |
ECM: |
extracellular matrix |
GAG: |
glycosaminoglycan |
IL-1: |
interleukin-1 |
INH: |
isoniazid |
LEDZ: |
liposome-encapsulated dextrazide |
MMP: |
matrix metalloproteinases |
MMT: |
macrophage-to-myofibroblast transformation |
Mtb: |
Mycobacterium tuberculosis |
OD: |
oxidized dextran |
PGs: |
proteoglycans |
RIF: |
rifampicin |
TB: |
tuberculosis |
TGF-β: |
transforming growth factor-β |
TIMPs: |
tissue inhibitors of metalloproteinases |
TNF: |
tumor necrosis factor |
α2-MG: |
α2-macroglobulin |
Declarations
Author contributions
LBK: Conceptualization, Methodology, Investigation, Writing—original draft, Writing—review & editing. ANP: Data curation, Formal analysis, Investigation, Project administration, Validation. Both authors read and approved the submitted version.
Conflicts of interest
The authors declare that they have no conflicts of interest.
Ethical approval
Not applicable.
Consent to participate
Not applicable.
Consent to publication
Not applicable.
Availability of data and materials
Not applicable.
Funding
This work was supported by budgetary funding for basic scientific research of Fundamental and Translational Medicine, Russia. The funder had no role in study design, data collection and analysis, decision to publish, or preparation of the manuscript.
Copyright
© The Author(s) 2023.