Abstract
The development of patient-specific prosthetics, medication administration, the manufacture of tissues and organs, and surgical planning have all benefited significantly from the use of three-dimensional (3D) printing during the past few decades. The enthusiasm for customized healthcare has increased because the United States of America launched its Precision Medicine Initiative in 2015. In a nutshell, the phrase “personalized medicine” refers to medical care that is tailored to the patient. Nevertheless, the biomedical materials utilized in 3D printing are often stable and can’t react or be adaptive and intelligent in the body’s interior environment. Ex-situ fabrication of these substances, which includes printing on a flat substrate before releasing it onto the target surface, may cause a discrepancy between the printed portion and the target areas. The 3D printing is one method that might be used to provide customized treatment. The four-dimensional (4D) printing is developed while employing components that can be tweaked with stimulation. Several researchers have been looking at a new area recently that blends medicines with 3D and 4D printing. The development of 4D printing overcomes a number of these issues and creates a promising future for the biomedical industry. Smart materials that have been pre-programmed can be used in 4D printing to create structures that react interactively to outside stimuli. Despite these benefits, dynamic materials created using 4D technology remain in their development. As a result, several ideas for pharmaceutical products and formulas that may be customized and printed have emerged. Furthermore, Spritam®, the first medicine produced by 3D printing, has indeed reached a medical facility. This paper offers a summary of several 3D and 4D printing technologies and how they are used in the pharmaceutical industry for customized medicine and drug delivery systems.
Keywords
3D printing, 4D printing, smart material, stereolithography, pharmaceutical, biomedicalIntroduction
Smart materials, also known as responsive or adaptive materials, are created materials with the capacity to continuously respond to environmental cues, altering their characteristics and functionalities for a particular demand or use [1–6]. These substances often react to stimuli including heat, ionic strength, pH, electromagnetism, light, humidity, and mechanical pressure [7]. They can also carry out living-like tasks such as self-assemble, self-healing, self-evolution, shape memory, detecting, and triggering [8–11]. Investigators have been compelled to create various techniques for behavior improvement and functionality in connection to certain purposes through the development and testing of structural systems. Due to the inherent mechanical and control limits of such machinery, smart materials have outpaced traditional production, becoming more complicated in terms of topologies, nano- and micro-structures, physical-chemical, as well as mechanical aspects [12].
A more recent version of “dimensional printing” known as “four-dimensional (4D) printing” has been made possible by recent developments in three-dimensional (3D) printable advanced materials. The concept of 4D printing acknowledges time as a fourth dimension and enables printed structures to modify their structural morphology in response to any outside input [13–14]. Excipients manufacturing, also known as 3D printing, has emerged in recent years as a versatile technique and a priceless replacement for conventional manufacturing for the construction of multiple substances through such a layer-by-layer methodology, resulting in the development of various types of medical technology, wearable devices, scaffolds, actuators, soft robotics, and flexible electronics [15–24].
However, personalized medicine does come with certain challenges. Major challenges are the production of these personalized medicines, which raises questions like: How can they be tailored to meet individual needs? How much time does it take to make a personalized therapeutic, and how can they be made routinely?
Here, the advancement of pharmaceuticals may benefit from the use of 3D and 4D printing. This cutting-edge technology was initially presented in 1986 by Hull [25], and it allows for the printing of 3D objects with different geometrical by depositing a variety of (bio)materials. Being a cutting-edge technique for tissue engineering, organ manufacturing, regeneration of drugs, and medication administration, 3D printing has been helpful in the biomedical field (Figure 1) [26]. Moreover, the ability to create patient-specific tailored orthotics, prostheses, craniofacial implantation, and medical equipment using personal information made 3D printing a significant draw in this sector [27]. It should be stressed that 3D printing has a substantial effect on medical learning and planning of surgery because 3D anatomical designs could be printed by accurately recreating the infinitesimal anatomical landmarks of body tissues. This has a favorable influence on the total time needed for the surgical procedure, its effectiveness, precision, and achievement, and could also be used to recruit young surgeons [28–29].
The creation of structures using 4D printing technology is possible since these structures may change over time in terms of shape, property, and functioning [30]. The capacity to self-assemble, self-repair, and multitasking is just a few of the amazing properties of 4D printed products. The technique became more logical as a result of time constraints and printer independence.
The planned activities and intellectual sensitivity of 4D material printing enable reactions to stimuli such as heat, light, pH, magnetic fields, etc. [31]. This new concept is built on three components: a smart material, a smart machine, and a geometric “program” [32]. Tibbits’ team [33] used a StratasysTM Connex 500 printer and a rigid plastic foundation with a soft component that extended when exposed to water to build a series of dynamic primitives (ring stretching, linear stretching, and folding). The expandable substance was a cross-linked hydrophilic polymer that, upon contact with water, transformed into a hydrogel and underwent a significant volume increase of up to 200% [34]. A bioinspired 4D printed hydrogel capsule for intelligently controlled medication release was created by Zu et al. [35].
In the pharmaceutical industry, wherein tissues and organs are by nature multifaceted and multipurpose settings, the creation of substances that are dynamic and biocompatible after printing is the next hurdle. Another significant problem of 3D and 4D printing is that intended objects are often produced on a flat, plane substrate first (ex-situ printing), and afterwards moved to the desired non-planar regions, like those found in the human body. Therefore, the printing procedure is fully deterministic, with limited “real-time knowledge” of the target geometry-except through computed tomography or laser scanning-thus leading to a possible mismatch between the printed part and target surfaces.
In this short review, a brief introduction to 3D and 4D printing as an intriguing method for creating cutting-edge smart materials for the development of medications. The most recent advances in 3D and 4D printing are then discussed, especially as they pertain to the pharmaceutical sector.
3D printing
Rapid prototyping or excipients manufacturing, both of which are terms used to describe 3D printing, are crucial in the development of medicines since they allow for the production of scaffolds that can either fix or substitute traditional modes of distribution (Figure 2). Using a bottom-up strategy is 3D printing. The cutting of magnetic resonance (MR) or digital picture of the product yields cross-sectional information that is used by a computer program to direct production. Because of this, manufacturing having greater structural intricacy may be done layer by layer utilising this method, which is especially useful for individual-specific implants [36]. The excipients manufacturing method known as selective laser sintering (SLS), fused deposition modelling (FDM) is one of the most widely utilised fast prototyping techniques, and light-assisted 3D-printing [stereolithography (SLA)] is the primary 3D printing subcategories that employ hard polymers for product production. Another type is bioprinting that employs polymeric hydrogels containing cells. The following list of concepts describes these strategies.
Conventional 3D printing technologies
The majority of traditional 3D printing methods use nozzle-based processes, wherein inks are printed one layer at a time under a spatial control that produces a certain design. The techniques comprise extrusion-based, near-field electrospinning (NFES), light-assisted, as well as inkjet-based ones [37–39].
FDM
One of the most popular quick prototyping methods is FDM, which was created and patented in 1989 by Crump [40]. Automotive, aviation, model creation for visualisation, design verification, and healthcare are just a few of the industries that have employed these technologies [41]. FDM works by first melting a thermoplastic polymer that has been added to the machine (in the type of a strand or particle) in the reactor vessel, then being ejected via a nozzle onto the platform and imprinted layer by layer to create a 3D shape. A computer program shifts the nozzle’s stance in the x-y plane during the manufacturing procedure to generate the design that best. As one layer is finished printing, the nozzle advances along the z-axis a predetermined length to print the subsequent layer. After the desired shape is produced, this procedure is repeated [42]. The size of the nozzle, print quality, angle, and spacing between fibres in succeeding layers, as well as the numbers of layers, all affect how finely detailed the finished version will be [43].
The fundamental advantage of FDM is its capacity for many mouldings with different materials. The method involves controlling nozzles carrying several thermoplastics such that they extrude consecutively, giving rise to a final shape with a variety of qualities. Additional benefits of the FDM include its speed, affordability, and simplicity [44]. As there is no solvent used in this procedure, organic solvents like acetone and chloroform that may be harmful to cells are eliminated [45]. The technology’s drawback is the dearth of components that are pharmaceutical grade and biocompatible and can be used as thermoplastics. Additionally, it is difficult to find materials with the proper melt viscosity, which should be high enough to deposit and low enough to extrude [46].
Light‑assisted 3D-printing (SLA)
Millions of micromirrors are controlled by a computerised micromirror arrangement in light-assisted 3D modelling, also known as SLA, so attempt to crosslink a photo-polymerizable pigment into the required shape [47]. Many complicated biological constructions may be swiftly printed using this technique, but only when using photocrosslinkable dyes [48]. Its rapid printing velocity of 200–1,600 mm/s, great clarity (depending on the measurement of the micromirrors), and good cell survival are its key benefits. Moreover, medications may be included with the ink before printing, resulting in matrix inclusion and regulated medication release. The main constraint is a smaller variety of inks that almost always depend on UV-crosslinking, perhaps putting cancer development at risk due to reactive oxygen moiety production [49].
SLS
The excipients manufacturing method known as SLS was created and trademarked in 1989 by Deckard [50]. This method involves the employment of laser light as the power source to melt a thin coating of powdered substance (metals, ceramics, and thermoplastic polymeric materials) that has been spread out in the shape of a powder bed. The computer program instructs the beam to ignite the substance and weld it together to create the two-dimensional (2D) form. A fresh coating of powder is disseminated on the top of the station by a piston to sinter the subsequent layer after the created platform has moved down 1 level of thickness following producing a layer. Until the last component is created, this process is carried out [51]. Excess material is cleaned when the fabrication is finished using a brushing or pressurized air. The ability to fabricate massive, intricate scaffolds is a benefit of SLS. An additional benefit is the fact that the sintering item is situated in firm powder particles and a protective layer is not required, SLS doesn’t really require additional support networks throughout the manufacturing process. Like FDM, SLS is a solvent-free manufacturing technique; therefore, the produced object is devoid of any solvent residue. Since spherical particles are fused to generate the product, this ultimately produces a rough texture; the major drawback of SLS is the fact that the item surface is just not clean and requires polishing [52].
3D printing based on extrusion
By forcing ink through with a tiny nozzle with force or other pressure changes, extrusion-based 3D printing generates an ongoing filament that enables layer by layer ink layering [53]. Using FDM and a temperature-controlled extruder, thermoplastic materials may be printed into the desired shape. In contrast to inkjet-based printing, extrusion-based 3D printing maintains a continuous flow and may, as a result, smoothly print inks onto such a surface. It is possible to add a pharmaceutical to the ink before printing, allowing for the drug’s continual release through microbial oxidation. On the other side, the extruder printing pace is rather slow (10–50 m/s), which makes larger constructions less feasible [54].
3D printing with inkjet
The Hewlett-Packard Corporation created the inkjet which was the initial excipients manufacturing printing technology in the 1970s as just a 2D printing technique. Eventually, in 1992, a cylinder as well as an elevating station that can travel along the z-axis was coupled towards this technology, and a 3D printing device was constructed [55]. For uses in tissue engineering, heat, as well as piezoelectric inkjet printers, are increasingly employed. A prepolymer mixture called ink, which could also comprise cells, is put in an ink cartridge for thermal inkjet printing. Little air bubbles produced by heating in the printing face serve to expel the ink droplets from the cartridge once it is inserted into the computer-controlled printer head. Ink thickness, the amplitude of the switching cycle, and the gradients of the implemented temperature may all influence the size of the droplets [56]. The piezoelectric inkjet printer’s operating system relies on utilizing suitable possibilities for the printer’s piezoelectric material, which produces the pressure required to expel the ink droplets out of the nozzle. The quick manufacturing and low cost of the gadget are the main benefits of inkjet printing [16].
3D printing using a laser
Another printing technology that utilizes a pulsed laser beam, a donating layer, and a receiver layer is laser-assisted 3D printing. The idea behind this technique is that ink is put on top of a ribbon with a very thin coating that absorbs energy. The ribbon is positioned parallel to the receptor. A vapor bubble is created when the pulsing laser source is concentrated on the light absorber material. This bubble exerts pressure that causes the ink to distort and produce droplets. Such cell-loaded hydrogel drops are sent in the direction of the sensor, where they’re gathered and crosslinked [57]. Due to the lack of a nozzle and the nonintrusive printing method used with this technology, it has the benefits of not clogging and not mechanically stressing the cells. They all improve cell viability. But, relative to certain other printers, this technological system seems to be more costly [58].
New methods for 3D printing
By merging sophisticated biomaterials with traditional 3D printing, new 3D printing methods seek to overcome their geometrical limits. For instance, utilizing the mini-tissue technique, multi-material 3D printing, which typically uses an extruder 3D printer, may create detailed constructs that mimic real tissues. Cluttering and slow printing speed, nevertheless, hinder this strategy [59]. Others here have created a method of 3D printing using multiple materials with light assistance that produces accurate architectural details. The main benefits associated with this method are the reduced mixing of various inks and the wide range of high-precision constructions; nevertheless, the disadvantages are scale-up, mechanization, resolution, and ink choice [60]. A micro-extrusion printer used for integrated 3D printing extrudes the required design into a printing fluid intended as a sacrifice. After cross-linking the appropriate printed build, the fatal bath is eliminated. Less geometrical limitations can be placed on the finished build as a result. This method’s primary drawbacks are its slow printing velocity and the need for an appropriate sacrificial print bath [61].
4D printing
According to the stimulus available in the milieu, additive-produced structures made of intelligent (smart) substances can self-modify into a specified shape or perform a predetermined purpose (Figure 3); these techniques are referred to as “4D printing” [33, 62]. The very same advanced manufacturing processes and tools covered previously in the 3D printing segment are used in 4D fabrication. The kind of substance used determines the fundamental distinction between 3D and 4D printing. A 3D printed object must have at least one intelligent activity, including such “composite materials” or “self-actuation,” to be classified as a 4D printed object [63]. Compared to 3D printing, 4D printers provide several benefits. The inclusion of the fourth dimension, duration, in the 3D structure allows for both temporal as well as spatial regulation of the manufactured good. By producing buildings that are dynamic and animated, 4D printing solves one of the main problems with 3D printing. Most frequently, “materials that demonstrate alterations to their physical or chemical characteristics in a regulated and useful manner following exposure to an environmental stimulation, including such temperature, humidity, light, magnetic field, or pH,” are known as intelligent components. In reaction to the above forms of inputs, a 4D printed product may alter its shape, color, functionality, or other chemical or physical attributes. Due to the intellect of the substance, the fully programmable condition and functionality of the 4D printed products remove the requirement for additional post-processing equipment or procedures, shorten manufacturing time, and in some situations may also help with the application procedure [33]. For instance, dynamic reaction after implant allows contouring smart scaffolding that displays compaction before in vivo application to be employed in non-invasive treatments and self-assemble to the necessary complicated shape [64].
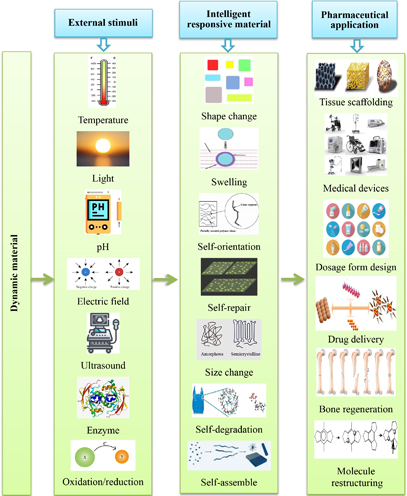
A schematic showing the many stimuli and reactions, including swelling, self-assemble, self-repair, and the potential for usage in pharmaceutical applications, are shown for smart materials
Aspects affecting 4D printing
The kind of added fabrication technique, the responsive substance, the kind of stimuli, the interaction process between both the stimulation and the content, and the mathematical modelling of the material transformation are the five primary aspects that affect the operation of 4D printing.
Applications of 3D printing
Uses for dermal applications
Transdermal drugs have been around for a while and offer several benefits, including being simple to administer, non-invasive, painless, and excellent for self-administration. Nonetheless, the stratum corneum’s impermeability renders pharmaceutical molecule permeation over the skin impossible without injections [65]. Hence, from the middle of the 1990s, the manufacture of high-resolution microneedle coverings with diameters that vary from 150 μm to 200 μm has grown into a popular study area. Nowadays, scientists may create whole microneedle patches using a variety of 3D printing processes, or they can cover already produced patches with the correct medication solution.
Uses for bone tissue engineering
A tissue with strong mechanical toughness and mineralization is bone. As a result, it is necessary to improve the mechanical characteristics of the printed polymers to better mimic those of bone tissue. Several types of research have been published in the literature that demonstrates the creation of 3D printed scaffolding for tissue engineering of bones. The engineering of bone tissue techniques employs a variety of types of cells. Mesenchymal stem cells made up of bone marrow or adipocytes constitute the most popular ones among all [66]. They are the best cell type to examine to regenerate bone because of their strong potential to develop into bone cells.
Oral medication formulations produced via 3D printing
Compared to conventional methods, 3D printing offers significantly greater operational flexibility for the production of tablet medications. More options are now available than with basic tablet compression because of its ability to create sophisticated tablets with the instant release, staggered release, prolonged release, pulsatory release, and the incorporation of numerous medications designed for multiple patterns all in one tablet [67]. For instance, recently presented a unique tablet design of a tablet which boosted the quick release of the drug of 3D printed tablets. The polymethacrylate tablets have internal passageways with specified widths, lengths, and arrangements. These canals raise the surface-to-volume ratio of the tablet, enabling a quicker rapid drug release. The precision of the channels was impacted by the 3D printing method utilized, FDM. Greater quality would make the printed structure more complicated and have a greater capacity for drug loading, which may let the drug release be more carefully regulated [68].
Personalized medicine dosage using 3D printed structures
Personalized medicine, which has tougher criteria for sustained release and complicated geometries, may be produced via 3D printing. It has previously been used to customize medication’s size, dose, form, and even color. It has also been used in a variety of ways, such as vaginal rings, tablets, and microneedle panels [69–70]. Using 3D printing, therapies may be customized to the patient’s condition. To improve the taste of the drugs and, consequently, the adherence of young patients, Scoutaris et al. [71] designed chewable candy-like compositions that could be 3D printed and were based on Starmix® candies. The tablets might be printed in a variety of forms, such as hearts, lions, bottles, and bears, and they increased the flavor (reduced bitterness). Furthermore, drug-release tests revealed that drugs dissolve after 60 minutes of ingestion [72].
Vascular tissue treatment via 3D printing
An incredibly crucial component of tissue repair is vascularization, thus 3D printing brings vascularization methodologies and contributes its benefits to produce vasculature and, as a result, healthier vascularized constructions [73]. Although co-polymers of poly(propylene fumarate)-co-poly(caprolactone) materials may be printed using SLA as well as inkjet printing, the most common uses currently concentrate on bioprinting. The building structure also contains fibroblasts and cells of smooth muscles. In certain experiments, spheroids and multicellular aggregation were combined to create scaffold-free constructions. Mice were used for testing 3D printed vasculature grafts consisting of Matrigel and endothelial progenitor cells [74]. These investigations all yielded encouraging vascularization findings.
3D printed implants that release drugs
Typically, biomedical implants are placed in the body to partially or completely restore the functions of a missing biological tissue, considerably boosting the patient’s quality of life. Implants with controlled drug release and site selection are now available as the implant business has evolved towards personalisation and customisation. Using a traditional production process, it is challenging to meet the complicated design and specifications of these implants. As a result, implantable medication delivery systems have been created during the past ten years using 3D printing, which has great potential in modern medicine. One indication of this is the growing preference among doctors for local delivery of chemotherapeutic drugs, which results in decreased systemic blood amount of drug, fewer side effects, and higher antitumor activity [75–76].
3D printing in nerve tissue treatment
The anisotropic alignment of the nerve fibers causes the directional (uniaxial) structure of nerve tissue. After such a lesion, a severed nerve’s proximal and distal terminals are brought together using nerve leads. Moreover, they may be created via 3D printing to offer patient-specific structures with such a sophisticated internal design. Devices for nerve tissue engineering are typically made using a variety of design and manufacturing techniques, notably SLA, inkjet printing, FDM, and bioprinting. Moreover, constructions for nerve tissue engineering purposes were printed using fibrin, gellan gum, collagen, carboxymethyl chitosan, polyurethane, and agarose [77–78].
Uses of 4D printing in medicine delivery
Numerous research investigations in the field of biomedicine describe the use of 4D printing to design tissues and organs. The printing of cell-filled constructs, self-folding tubes, including biosensors is a few examples of what is known as 4D bioprinting. Drug administration systems created using 4D printing are a relatively new notion, and while some studies did not specifically reference 4D printing, their models did contain certain 4D printing elements. This part will address what may be classified as 4D printing in the drug delivery sector and its application programs since the idea of 4D printing are previously defined in bioprinting technology [79].
4D printed hydrogels as drug delivery systems
Hydrogels should be mentioned as the initial application. They have the special ability to absorb and hold a lot of liquid, whether they are constructed of polymers that are either synthetic or natural. Hydrogels have also been discovered use as drug delivery methods because of their biodegradability and biocompatibility. About the fourth dimension of hydrogels, particular hydrogels’ stimulus sensitivity makes them great prospects for 4D drug delivery methods and may help with medications’ sustained release (Figure 4). The 4D printing of hydrogels has been demonstrated in several types of research using a variety of methods and bio-ink formulas. Few have, nevertheless, actually used them as 4D printed medicine delivery devices [80].
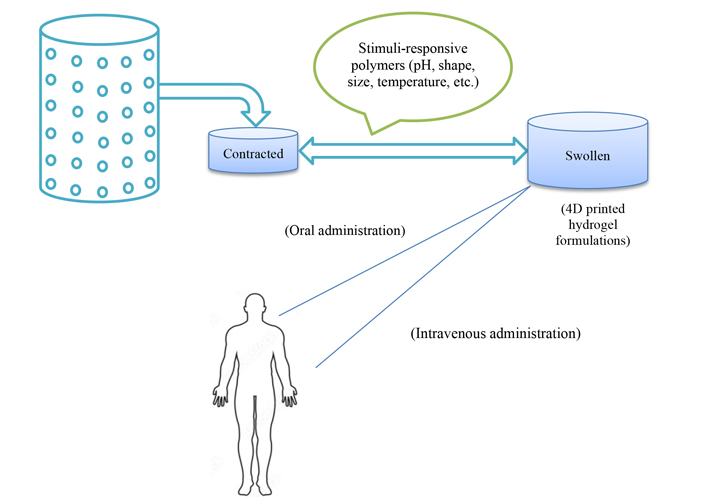
A summary of the uses of stimuli-responsive hydrogels in 4D printed medication delivery systems
Oral formulations created via 4D printing
An outstanding shape-memory expandable gastroretentive drug delivery system (SMX GRDDS) device was created by Melocchi et al. [81]. FDM was carried out using a Kloner3D® 240 Twin with a 0.5 mm nozzle. The prototype medicine used in the printing process was the xanthine oxidase antagonist allopurinol (ALP), as well as the printed material was a combination of poly(vinyl alcohol) (PVA) and glycerol (GLY). A circular helix-shaped construction with a width of 15 mm and a height of 17 mm was printed using the combination PVA05GLY-ALP at a rate of 23 mm/s. The crew demonstrated how they could print various forms utilizing printing moulds. The SMX GRDDS in its temporary structure was put into a capsule intended for oral consumption. The printed structure was exposed to 0.1 mol/L HCl at 37℃, which replicates the acidic environment in the gastrointestinal, and the capsule instantly started to deteriorate. Irrespective of the printed form, the technology demonstrated a continuous release of the medication over the period of 2 h [81].
Microneedles produced via 4D printing
Han et al. [82] have demonstrated the use of innovative 4D printing to create a microneedle matrix that improved tissue adherence. A combination of Sudan I as a light absorber, phenylbis(2,4,6-trimethylbenzoyl), and phosgene oxide as a photoinitiator was 3D-printed by the team using a specially constructed projections microstereolithography. Han et al. [82] imprinted horizontal curving prongs on the microneedles which might flex into a curved geometry following post-printing dissolution to improve tissue adherence again for the microneedle matrix. Relative to a needle lacking barbs, the barbs caused skin adherence to rise 18-fold. Adhesion is thought to be crucial when a cutaneous drug-eluting technology is finally releasing its medicine over time. A model medication, the luminous rhodamine B compound, was used to show transdermal drug delivery [82].
Drug-eluting 4D printing-implants
Stimuli-responsive implants for medication administration have a wide range of uses. Some teams have developed implants that potentially serve as medication delivery devices using cutting-edge 4D printing methods [83–84]. Few have successfully placed a (model) medication within a 3D-printed implant that responds to inputs. A new thermoplastic polyurethane (TPU) was introduced by Salimi et al. [85] and employed as a framework enabling drug-eluting implants. The team employed UV light-emitting diodes curing equipment as well as a pressurized extruded bioprinter (CELLINK, Sweden) to create a bar-shaped implant using a combination of TPU and poly(ethylene glycol) (PEG). As a prototype medication, paracetamol (16%) was inserted into the bar-shaped implant. The implant showed good biocompatibility but no discernible cytotoxic effect in the mouse fibroblast cell line. The substance was indeed flexible but rigid and provided a regulated delivery of paracetamol. Even though the implant exhibited certain advantageous characteristics, Salimi et al. [85] observed distortion of the implant before and during drug release, suggesting that the phase change materials of TPU should be adjusted.
Magnetically activated drug delivery system
A 4D printed material may be made using magneto-restrictive elements that are triggered by a magnetic field from the outside, which may help with targeted medicine administration for the least amount of adverse effects and the best dosage. For instance, using a traditional lithographic approach, Gazzaniga et al. [86] created a formulation using a hydrogel bilayer. One of the layers had pH-responsive characteristics, which helped with drug release by altering the layer’s shape when exposed to a certain pH. A different layer, however, included iron oxide particles that allowed the formulation to be magnetically directed and guaranteed site-specific medication delivery. This specific discovery can be applied to the targeted administration of anti-cancer medications. Low oxygen partial pressure, a particular pH in tumor tissue, and the microenvironment of the tumor tissue can all stimulate the release of drugs [87].
pH-guided drug delivery system
It is conceivable to create a pH-directed controlled release medication delivery system employing smart substances that react particularly to pH change. A pH-sensitive formulation was used by Mathur et al. [88] to create a mucoadhesive system for drug delivery that changes form and adheres to the gut wall as it enters the small intestine (pH 6.5). These self-folding formulations showed raised drug absorption via the mucosal epithelium, decreased drug contact with intestinal fluid, and an extended residence time [88].
Conclusions
The creation of products in the healthcare and pharmaceutical industries now uses 3D printing technology. These advanced additive manufacturing techniques use computer-aided design and computer software to quickly and affordably produce compositions with complicated shapes. With the use of this method, the necessity for time-consuming, multi-step processes in the creation of various dosage forms was reduced. Spritam® which was released to the public in 2015 was the first medicine made via 3D bioprinting to get the US Food and Drug Administration approval. Over the past twenty years, several methods have been established, including inkjet printing, SLS, powder bed printing, SLA, pressure-assisted microsyringes, and fused accumulation simulation. Nevertheless, a lot of these approaches are still constrained by low productivity when compared to conventional approaches to medicine manufacturing. Scaling up is therefore crucial for closing the gap between the development and commercialization of 3D printed medication formulations. The biomaterials inherently impose further restrictions on the use of 3D bioprinting in the pharmaceutical business. The elevated temperatures may not be the best for the medicine formulations included inside the biomaterial, which are frequently required in 3D bioprinting or biomaterial sterilization procedures.
A unique idea called 4D printing enables the development of the technology for 3D printing to create intelligent, time-dependent goods. The goal of this notion is to create items that can self-assemble, self-repair, and have many uses. The pharmaceutical and biological sectors hold greater promise for its use. Employing this sort of technology, it can be done to create unique smart pharmaceutical formulations. It still needs further study on software, mathematical modelling, mechanical, and chemical challenges because it is still in the early stages of development. There are a few self-assemble and multi-responsive substances that have been looked into for 4D printing. It is necessary to do a more in-depth study to identify raw materials that may serve as the input for 4D printing methods. In the event of biomedical usage, the printed product’s biocompatibility, biodegradability, and in situ milieu should all be taken into account. To produce 4D printed biomedical devices that may be used in clinical settings, scientists are striving very hard. This study can be sure that 4D printing will revolutionise the industrial sector and unleash a multitude of hitherto undiscovered aspects that will contribute to making our future more intelligent.
Abbreviations
3D: |
three-dimensional |
4D: |
four-dimensional |
FDM: |
fused deposition modelling |
SLA: |
stereolithography |
SLS: |
selective laser sintering |
TPU: |
thermoplastic polyurethane |
Declarations
Acknowledgments
The authors are highly thankful to ShriRam College of Pharmacy, Banmore, Morena, for providing necessary facilities and encouragement.
Author contributions
PS: Writing—original draft. VJ: Writing—review & editing, Supervision. PS and VJ read and approved the final manuscript.
Conflicts of interest
The authors declare that they have no conflicts of interest.
Ethical approval
Not applicable.
Consent to participate
Not applicable.
Consent to publication
Not applicable.
Availability of data and materials
Not applicable.
Funding
Not applicable.
Copyright
© The Author(s) 2023.