Abstract
Aim:
The development of a collaborative strategy with improved efficacy holds great promise in tumor treatment. This study aims to develop an effective collaborative strategy based on functionalized mesoporous polydopamine (MPDA) nanocomposites for killing tumor cells.
Methods:
MPDA nanoparticles were synthesized and functionalized with camptothecin (CPT) payload and manganese dioxide (MnO2) coating to construct MPDA-CPT-MnO2 nanocomposites.
Results:
When uptaken by tumor cells, the nanocomposites can degrade to produce O2, release CPT, and generate manganese (Mn2+) under the stimulation of hydrogen peroxide (H2O2) and acid. The released CPT and Mn2+ can act as chemotherapeutic drug and Fenton-like agent, respectively. Abundant reactive oxygen species (ROS) are generated in 4T1 tumor cells through an Mn2+-mediated Fenton-like reaction. After that, the generated Mn4+ can react with glutathione (GSH) through redox reaction to produce Mn2+ and deplete GSH, disrupting the reducing capacity and benefiting the production of ROS in tumor cells. Under laser irradiation, the nanocomposites can generate hyperthermia to promote the production of ROS.
Conclusions:
The developed MPDA-CPT-MnO2 nanocomposites can kill tumor cells through collaborative chemo/photothermal/chemodynamic therapy (CDT).
Keywords
Mesoporous polydopamine, chemotherapy, photothermal therapy, chemodynamic therapy, tumor treatmentIntroduction
Photothermal therapy (PTT) is a type of tumor therapeutic method that involves artificially elevating local tissue temperature under near-infrared (NIR) light irradiation [1–6]. Due to its high inherent specificity and minimal intrusive burden, PTT is appealing in comparison to conventional tumor treatment techniques such as surgery, radiation, and chemotherapy (CT) [7, 8]. However, its treatment efficacy is limited by the conversion efficiency of photothermal agents (PTAs) and the penetration depth of NIR light [9, 10]. Combining PTT with other treatment techniques to construct multimodal treatment strategy holds great promise [11, 12]. For example, various chemotherapeutic drug delivery systems based on PTAs have been developed, showing synergistic effects between PTT and CT [13–19].
Among extensively explored chemotherapeutic drugs, camptothecin (CPT) is a hydrophobic plant alkaloid extracted from Camptotheca acuminate, which can damage the DNA of tumor cells [20–22]. CPT inhibits topoisomerase I and forms irreversible covalent compounds with it, leading to DNA double-strand breaks and inducing cell apoptosis [23]. Nonetheless, its clinical application has been hampered by poor water solubility, high systemic toxicity, hydrolysis inactivation, and so on [24, 25]. Therefore, it is necessary to design a drug delivery system to deliver CPT to tumor cells specifically [26, 27].
The nanocomposites that own photothermal conversion performance and drug loading capacity hold the potential to develop a synergistic CT/PTT strategy. As synthetic analogs of the naturally occurring eumelanin, mesoporous polydopamine (MPDA) is a kind of popular drug carrier due to its simple preparation method, good biocompatibility, high drug loading efficiency, and easy surface modification [28, 29]. Furthermore, the great photothermal conversion ability of MPDA makes it an excellent candidate for PTT [30, 31]. When delivering drugs, the pores on the MPDA surface should be blocked to avoid the leakage of drugs [32, 33]. Manganese dioxide (MnO2) nanostructures own good pH-responsive performance and can be degraded under acid stimulation, showing great potential in tumor therapy [34–37]. At a low pH condition, the MnO2 nanostructure can be rapidly broken, acting as a pH-responsive drug carrier or sealer for mesoporous nanomaterials [38, 39]. The unique reaction between MnO2 and hydrogen peroxide (H2O2) can generate O2, alleviating the drug resistance of tumor cells to enhance the CT effect [40, 41]. In addition, manganese (Mn2+) is a Fenton-like agent that can catalyze H2O2 to generate hydroxyl radicals (•OH) for tumor chemodynamic therapy (CDT), which is a promising tumor therapy method [42–44]. The formation of Mn2+(HCO3–)2 complex is essential for Mn2+ ions to play the role of Fenton-like agents [45].
Herein, an effective collaborative strategy based on functionalized MPDA nanocomposites was designed and developed for killing tumor cells (Figure 1). MPDA nanoparticles were prepared as the carrier to load the chemotherapeutic drug CPT and then coated with MnO2 layer on the surface through the in situ redox reaction between KMnO4 and MPDA, finally obtaining MPDA-CPT-MnO2 nanocomposites. When up-taken by tumor cells, the outer layer of MnO2 reacts with H2O2 to generate O2 and Mn2+. In the presence of HCO3–, Mn2+ undergoes a Fenton-like reaction to produce •OH [46], which breaks the redox balance to cause tumor cell damage. After the MnO2 coating is destroyed, the chemotherapeutic drug CPT is released from MPDA nanoparticles to cause cell apoptosis. Under laser irradiation, hyperthermia is generated by MPDA for PTT. Compared with the reported studies, this study developed a kind of rationally designed nanocomposites to realize collaborative CT/PTT/CDT against tumor cells.
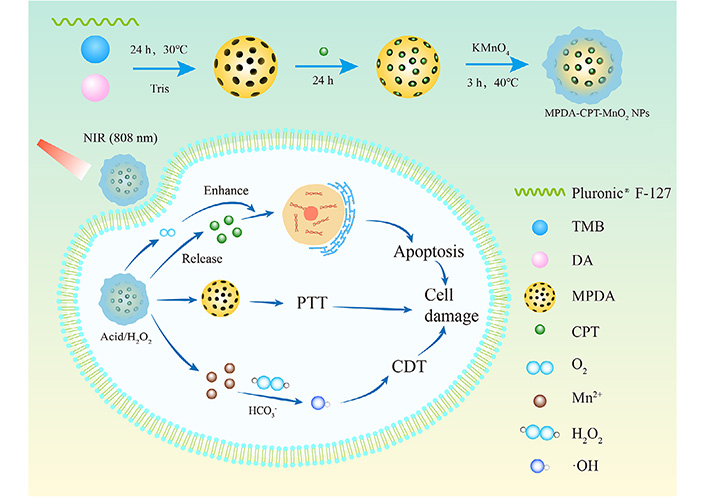
Schematic representation of the formation process of MPDA-CPT-MnO2 nanocomposites and the mechanism for tumor cell killing through synergistic CT/PTT/CDT strategy. DA: dopamine; KMnO4: potassium permanganate; NPs: nanoparticles; Pluronic® F-127: poly(ethylene glycol)-block-poly(propylene glycol)-block-poly(ethylene glycol); TMB: 1,3,5-trimethylbenzene; →: reaction process
Materials and methods
Materials
Tris (hydroxymethyl) aminomethane (Tris, 99.9%, CAS: 77-86-1) was purchased from Damas-beta. Dopamine hydrochloride (98%, AR, CAS: 62-31-7) was purchased from Titan (Shanghai, China). TMB (AR, 97%, CAS:108-67-8), dimethyl sulfoxide (DMSO, GC, 99.8%, CAS:67-68-5), glutathione (GSH, CAS: 70-18-8), 5,5’-dithiobis (2-nitrobenzoic acid) (DTNB, CAS: 69-78-3), and methylene blue (MB, CAS: 61-73-4) were purchased from Aladdin Reagent (Shanghai, China). H2O2 (30%, CAS: 7722-84-1) and KMnO4 (CAS: 7722-64-7) were supplied by Chuandong Chemical Co., Ltd. (Chongqing, China). Phosphate buffer saline (PBS, Item No.: BF-0011, Beyotime, Shanghai, China), Pluronic® F-127, 5-hydroxyfluorescein resinamide (5-FAM, CAS: 76823-03-5), 2’7’-dichlorodihydrofluorescein diacetate (DCFH-DA, CAS: 4091-99-0), Cell Counting Kit-8 (CCK-8, Item No.: C0039), GSH detection kit (Item No.: S0053), H2O2 detection kit (Item No.: S0038), Hoechst 33342 (Item No.: C1025) were purchased from Beyotime (Shanghai, China). Annexin V-fluorescein isothiocyanate/propidium iodide (V-FITC/PI) apoptosis detection kit (Item No.: C1062M) was obtained from Solarbio (Beijing, China). Deionized (DI) water (CAS: 7732-18-5) was used in all preparation processes from the purification system (Synergy, Millipore, MA). Other materials include: TMB (CAS:108-67-8, Aladdin Reagent, Shanghai, China); CPT (CAS: 7689-03-4, Aladdin Reagent, Shanghai, China); potassium permanganate solution (CAS: 7722-64-7, Aladdin Reagent, Shanghai, China); portable dissolved oxygen meter (ST300D, OHAUS, Changzhou, China); dialysis bags (Item No.: MD1444, Yunaye Reagent, Shanghai, China); (UV-Vis)-NIR spectrophotometer (UV-1800, Shimadzu, Japan); well plates (Item No.: 11510, Titan, Shanghai, China); flow cytometry (NovoCyte TM 2060R, USA).
Preparation of MPDA-CPT-MnO2 nanocomposites
For the preparation of MPDA nanoparticles, 0.36 g of Pluronic® F-127 and 834 μL of TMB were dissolved in a mixture of H2O (65 mL) and ethanol (60 mL) under stirring. After 30 min, 90 mg of Tris and 60 mg of dopamine hydrochloride were added successively into the mixture. The reaction was performed for 24 h at 30°C and the product pellet was then isolated by centrifugation (8,000 rpm) and washed with ethanol five times.
For CPT loading, 1 mg/mL MPDA aqueous solution and 0.5 mg/mL CPT in DMSO solution were stirred for 24 h under dark conditions, and the product pellets were separated by centrifugation and washed twice with DI water. For MnO2 coating, 1 mg/mL MPDA-CPT aqueous solution and 0.1 mg/mL potassium permanganate solution were reacted in a water bath at 40°C for 3 h. The product pellets were separated by centrifugation and washed with DI water. The products were dispersed in an aqueous and stored at 4°C. For comparison, MPDA-MnO2 without CPT loading was also prepared using the same method.
Photothermal performance evaluation
A series of aqueous solutions of MPDA-CPT-MnO2 nanocomposites in a concentration gradient (0, 100, 200, 300, and 400 ppm) were irradiated with an 808 nm laser (5 min, 1.0 W/cm2) to record the temperature values. The aqueous solution of MPDA-CPT-MnO2 nanocomposites and MPDA nanoparticles (400 ppm) was irradiated with the same laser (10 min, 1.0 W/cm2) and cooled for 20 min. Their photothermal stability was also tested by six repeated cycles of laser on/off process.
O2 generation assay
To verify the O2 production capacity, H2O2 (100 mmol/L) was added to an aqueous solution of MPDA-CPT-MnO2 nanocomposites (0.2 mg/mL). The concentration of O2 generated was determined by a portable dissolved oxygen meter.
CPT release test
MPDA-CPT and MPDA-CPT-MnO2 nanocomposites (0.5 mg/mL, 2 mL) were packed into dialysis bags (molecular weight = 8,000–14,000) and immersed in the PBS (pH 6.4, 100 mL) at 37°C under the protection from light. At various time points, the dialysate (1 mL) was taken and the absorption at 369 nm was measured by spectrophotometer (UV-1800, Shimadzu). An equal volume of fresh buffer was replenished to maintain the total volume unchanged.
GSH consumption assay
The solutions of MPDA-CPT-MnO2 nanocomposites with concentrations of 0, 50, 100, 200, 300, and 400 ppm were added to the GSH PBS solution (1 mmol/L, pH 7.4). After reacted for 6 h, 0.9 mL of supernatant was collected and mixed with DTNB solution (50 μL, 5 mg/mL), which can act as the GSH probe [47]. After reacting for 30 min, the absorbance of the supernatant at 412 nm was measured by an ultraviolet-visible (UV-Vis)-NIR spectrophotometer.
•OH detection assay
The mixture of H2O2 (20 mmol/L), MB (0.1 mg/mL), and sodium bicarbonate (100 mmol/L, CAS: 144-55-8) was prepared. The solutions of MPDA-CPT-MnO2 nanocomposites with final concentrations at 0, 20, 40, 60, 80, and 100 ppm were added to the above mixtures and reacted for 1 h. Then, the mixture (1 mL) was centrifuged to obtain the supernatant for UV-Vis-NIR measurement to determine the absorbance at 664 nm.
Cellular uptake assay
4T1 cells were inoculated in 12-well plates (1.0 × 105 per well) and incubated for 12 h. After co-incubation of the cells with the obtained MPDA-CPT-MnO2 nanocomposites for 1, 3, and 6 h, the cells were washed three times with PBS. The cell uptake behavior was analyzed by flow cytometry (NovoCyte, ACEA). In addition, fluorescent images were captured by confocal laser scanning microscopy [(CLSM) 780, CarlZeiss, Germany].
In vitro cytotoxicity assay
4T1 cells were inoculated in 96-well plates (1.5 × 104 per well) and incubated for 12 h. The cells were then co-incubated with different products for 24 h. Afterward, the cells were washed 3 times with PBS and incubated with 100 μL of medium containing 10 μL of CCK-8 reagent for 30 min. Finally, cell viability was assessed and recorded by a microplate reader (SPARK 10M, Tecan). To study the PTT effect, the cells in corresponding groups were treated with laser irradiation (808 nm, 5 min, 1.0 W/cm2) and the cell viability was measured using the same method.
Intracellular H2O2 and GSH measurement
4T1 cells were inoculated in 6-well plates (5.0 × 105 per well) and incubated for 12 h. The cells were then co-incubated with PBS, MPDA, MPDA-MnO2, and MPDA-CPT-MnO2 for 6 h. The concentration of all the nanocomposites was kept at 200 ppm. After that, the cells were collected and washed with PBS. The intracellular H2O2 and GSH levels were tested using the H2O2 detection kit and GSH detection kit, respectively.
Intracellular reactive oxygen species detection
4T1 cells were inoculated in 12-well plates (1.0 × 105 per well) and incubated for 12 h. The cells were then co-incubated with different products (200 ppm) for 6 h. Afterward, the cells were washed 3 times with PBS and stained with DCFH-DA as a reactive oxygen species (ROS) probe for 30 min. Finally, fluorescent images were collected by CLSM.
Apoptosis assay
4T1 cells were inoculated in 12-well plates (1.0 × 105 per well) and incubated for 12 h. The cells were then co-incubated with different products (200 ppm) for 24 h. Afterward, the cells were washed 3 times with PBS. The cells were treated according to the apoptosis kit instructions and finally processed by flow cytometry.
Results
Characterization
Transmission electron microscopy (TEM) image revealed that MPDA showed a regular porous structure with a uniform diameter of around 200 nm (Figure 2a). After CPT loading and MnO2 coating, the porous structures were invisible, indicating the success of surface coating (Figure 2b). Energy dispersive spectrometer (EDS) mapping revealed the co-existence of C, O, and Mn elements in the formed nanocomposites (Figure 2c). The X-ray photoelectron spectroscopy (XPS) spectrum of Mn 2p displayed two peaks at 652.78 eV and 641.08 eV, corresponding to the Mn(IV) 2p1/2 and Mn(IV) 2p2/3 spin-orbit peaks of MnO2, respectively (Figure 2d). The content of Mn was measured by inductively coupled plasma-optical emission spectrometry (ICP-OES) to be 9.64% in the final MPDA-CPT-MnO2 nanocomposites. The Fourier transform infrared (FTIR) spectra of the obtained products demonstrated the successful loading of CPT and coating of the MnO2 shell on the MPDA surface (Figure 2e) [48, 49]. The UV-Vis spectrum of MPDA showed no obvious peak (Figure 2f). After CPT loading, a typical peak at 369 nm was detected, indicating the loading of the CPT drug. The loading content was calculated to be 21.4% according to its standard curve. The mean hydrodynamic diameter of MPDA was tested to be 210.5 nm with a polydispersity of 11.7, which increased to around 270.1 nm for the final MPDA-CPT-MnO2 nanocomposites (Figure 2g). In addition, the zeta potential of the products kept similar around –20.0 mV (Figure 2h). The formed MPDA-CPT-MnO2 nanocomposites could disperse well in DI water, PBS (pH 7.4), and fetal bovine serum (FBS) during 48 h, indicating their good stability under physiological conditions (Figure 2i).
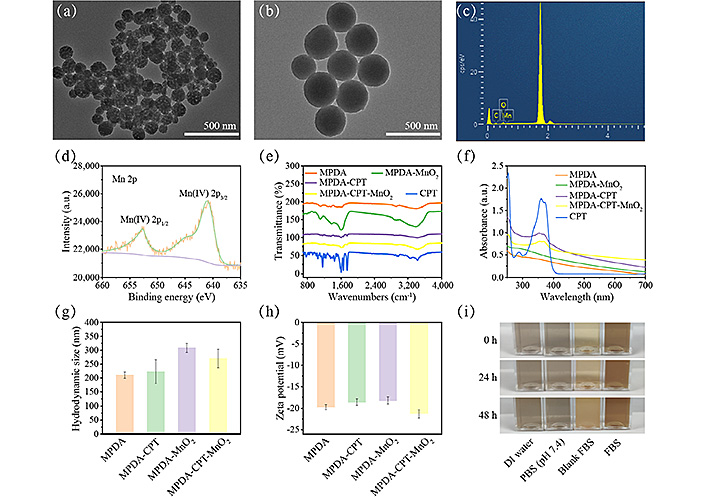
Synthesis and characterization of MPDA-CPT-MnO2 NPs. TEM images of (a) MPDA and (b) MPDA-CPT-MnO2; (c) EDS mapping analysis of MPDA-CPT-MnO2 nanocomposites; (d) the high-resolution XPS spectrum of Mn 2p in MPDA-CPT-MnO2 nanocomposites; (e) FTIR spectra of the obtained products and free CPT; (f) UV-Vis spectra; (g) hydrodynamic size; (h) zeta potential of MPDA, MPDA-CPT, MPDA-MnO2, and MPDA-CPT-MnO2; (i) the digital photos of MPDA-CPT-MnO2 nanocomposites when dispersed in DI water, PBS (pH 7.4), and FBS at 0, 24, and 48 h; CPS: counts per second
Photothermal performance evaluation
The solutions of MPDA-CPT-MnO2 nanocomposites displayed elevated temperature curves during 5 min laser irradiation, showing a positive correlation with the nanocomposite concentration (Figure 3a). For 400 ppm condition, the solution temperature increased from 25.1°C to 45.2°C after 5 min laser irradiation. The photothermal reproducibility of MPDA-CPT-MnO2 nanocomposites was assessed under 808 nm laser irradiation (Figure 3b). The photothermal conversion efficiencies of MPDA-CPT-MnO2 nanocomposites and MPDA nanoparticles were calculated by linear fitting calculations to be 31.5% and 32.3%, respectively (Figure 3c–f).
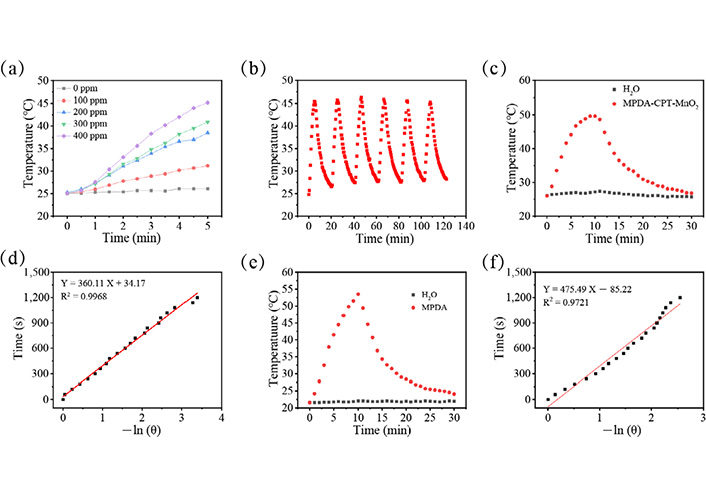
Photothermal performance evaluation. (a) Temperature data of MPDA-CPT-MnO2 nanocomposite solutions with different concentrations under laser irradiation; (b) the temperature curve of 400 ppm MPDA-CPT-MnO2 nanocomposite aqueous solution during six lasers on/off cycles; the heating-cooling curve of (c) 400 ppm MPDA-CPT-MnO2 nanocomposite aqueous solution; (d) was the linear time data versus –ln (θ) obtained from the cooling period in (c); (e) 400 ppm MPDA nanoparticle aqueous solution during 30 min; (f) were the linear time data versus –ln (θ) obtained from the cooling period in (e)
Stimuli-responsive performance and ROS production detection
As shown in Figure 4a, MPDA-CPT-MnO2 nanocomposites can react with H2O2 to produce O2, which can further alleviate the drug resistance of tumor cells. Then, the loaded CPT was released from the nanocomposites (Figure 4b). When compared to the un-coated nanocomposites (MPDA-CPT), the release of CPT from MPDA-CPT-MnO2 nanocomposites was postponed. The production of •OH was detected using MB as a probe. The characteristic absorption peak of MB was found to gradually disappear with the increase of nanocomposite concentration, and meanwhile, the color changed from blue to colorless (Figure 4c). DTNB was used as the probe to detect the depletion of GSH (Figure 4d). The characteristic peak was detected to decrease with the increase of nanocomposite concentration, demonstrating the redox reaction-caused GSH depletion.
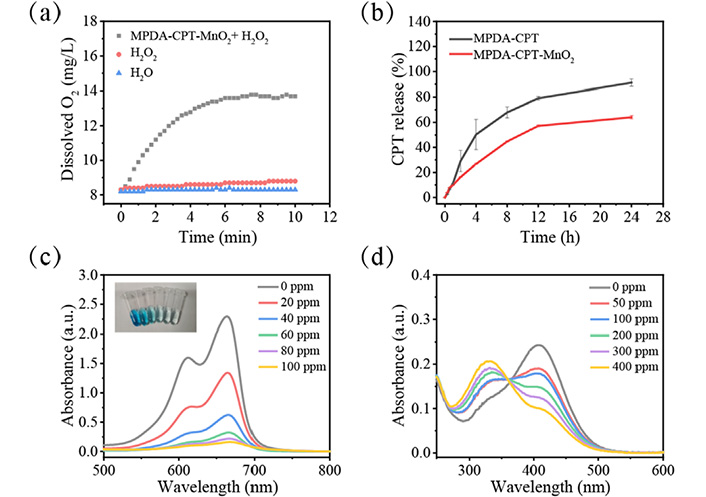
In vitro performance investigation. (a) O2 production curves of MPDA-CPT-MnO2 nanocomposites in the presence of H2O2 at room temperature; (b) CPT release curves from MPDA-CPT and MPDA-CPT-MnO2 nanocomposites in pH 6.4 buffer solution; (c) UV-Vis spectra and (inset) digital photo for •OH detection using MB as the probe in the presence of different concentrations of MPDA-CPT-MnO2 nanocomposites; (d) UV-Vis spectra for GSH detection using DTNB as the probe in the presence of different concentrations of MPDA-CPT-MnO2 nanocomposites
Cellular uptake
First, the cellular internalization ability of MPDA-CPT-MnO2 nanocomposites was characterized. The nanocomposites were fluorescence-labeled using 5-FAM and co-cultured with 4T1 cells. It was found that the fluorescence signal gradually increased with the nanocomposite concentration and incubation time (Figure 5a–c). As shown in the CLSM images, the cells at 6 h showed the strongest green fluorescence signal (Figure 5d).
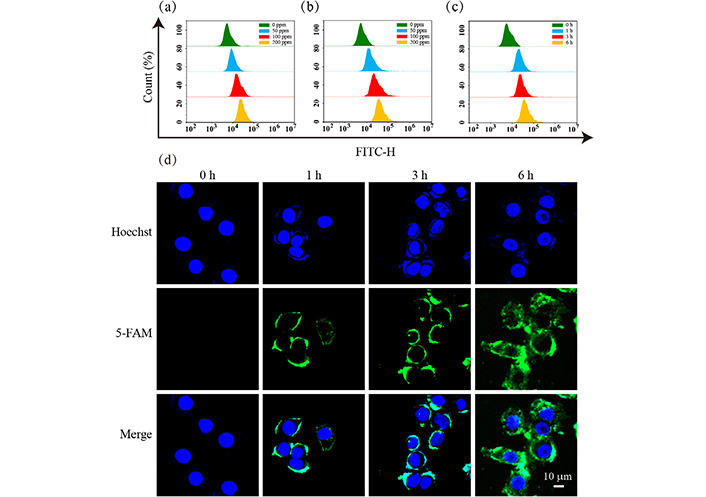
Cellular uptake performance. Flow cytometry curves of 4T1 cells after co-incubation with MPDA-CPT-MnO2 nanocomposites of different concentrations (0, 50, 100, and 200 ppm) for (a) 3 h and (b) 6 h; (c) flow cytometry curves of 4T1 cells after co-incubation with 200 ppm MPDA-CPT-MnO2 nanocomposites for different time points (0, 1, 3, and 6 h); (d) CLSM images of 4T1 cells after co-incubation with 200 ppm MPDA-CPT-MnO2 nanocomposites for different time points (0, 1, 3, and 6 h); H: height
Cell viability assay and mechanism exploration
As shown in Figure 6a, the cells in the MPDA group displayed similar viability data (0–200 ppm) to that of the control group, revealing the good cytocompatibility of the formed MPDA nanoparticles. A slight influence on cell viability was observed when the concentration of MPDA nanoparticles reached 300 ppm and above. The cells in the MPDA-MnO2 and MPDA-CPT groups decreased due to the therapeutic effect of CDT and CT, respectively. When treated with MPDA-CPT-MnO2 nanocomposites, the cell viability decreased significantly, which was further decreased under laser irradiation. At 300 ppm and 400 ppm conditions, the cell viability data in the MPDA-CPT-MnO2 plus laser group displayed great significant difference to all other groups.
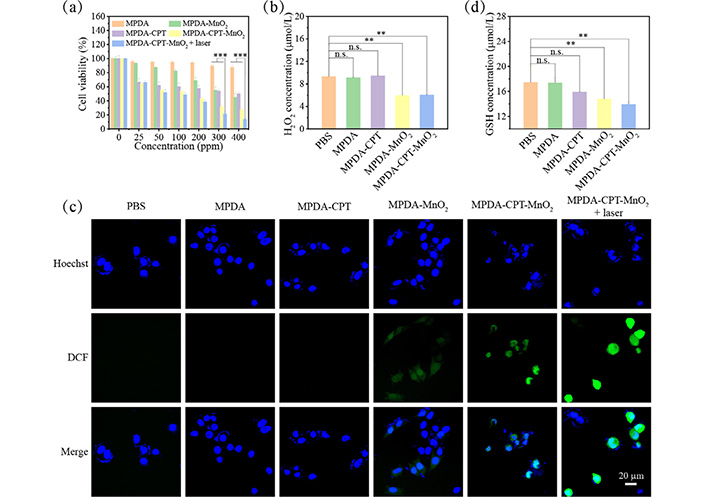
Intracellular performance evaluation. (a) 4T1 cell viability data after 24 h treatment with different samples without or with laser irradiation; (b) intracellular H2O2 level measurements after incubation with MPDA, MPDA-CPT, MPDA-MnO2, or MPDA-CPT-MnO2 nanocomposites; (c) DCFH-DA kit for the detection of intracellular ROS levels in 4T1 cells after different treatments; (d) intracellular GSH level measurements after incubation with MPDA, MPDA-CPT, MPDA-MnO2, or MPDA-CPT-MnO2 nanocomposites (*** P < 0.001 and ** P < 0.01) n.s.: non-significance; DCF: 2’7’-dichlorofluorescein
Then, the therapeutic mechanism was explored. The H2O2 levels in cells after treatments were shown in Figure 6b. Then, DCFH-DA was employed as a fluorescent indicator to measure the intracellular ROS level. The fluorescence images showed that 4T1 cells cultured with MPDA-MnO2 nanocomposites exhibited higher green fluorescence than that of the PBS group, demonstrating the production of ROS (Figure 6c). It was detected that the GSH level decreased significantly in the presence of MnO2 (Figure 6d).
In addition, the apoptosis percentages after different treatments were tested using an apoptosis kit (Figure 7). It was found that the apoptosis percentages in the MPDA-MnO2 and MPDA-CPT groups were 11.35% and 26.34%, respectively, which increased to 29.93% after MPDA-CPT-MnO2 nanocomposite treatment. The highest apoptosis percentage was detected to be 38.54% in the MPDA-CPT-MnO2 plus laser group.
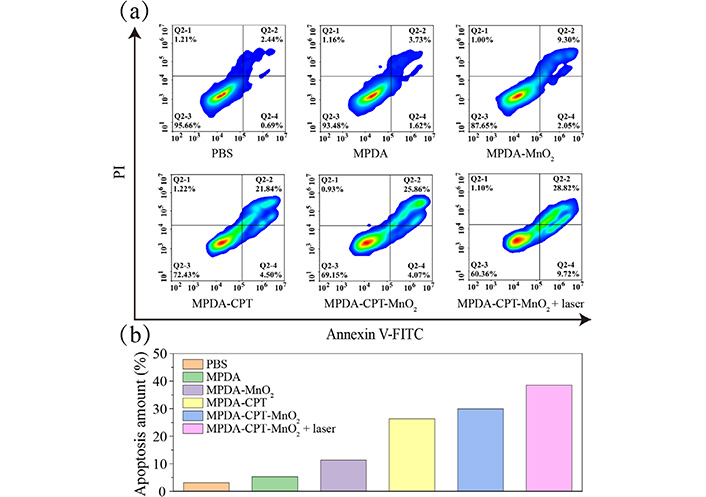
Apoptosis analysis. (a) Apoptosis data; (b) the corresponding quantitative histogram of 4T1 cells measured by flow cytometry after treatment with MPDA, MPDA-MnO2, MPDA-CPT, MPDA-CPT-MnO2, or MPDA-CPT-MnO2 nanocomposites. The corresponding group was laser irradiated
Discussion
MPDA nanoparticles were prepared by self-polymerization of dopamine hydrochloride using Pluronic® F-127 as surfactant and TMB as templating agent. MPDA nanoparticles own abundant surface phenyls, amino, and hydroxyl groups, endowing them with the excellent capability to load various chemical drugs through π-π stacking and/or hydrogen bond interaction [50]. The MnO2 coating layer displayed negligible influence on the absorption spectrum. Through characterization, the MPDA-CPT-MnO2 nanocomposites were successfully formed with good stability.
The photothermal property of MPDA-CPT-MnO2 nanocomposites at different concentrations was investigated using an 808 nm laser. They showed good photothermal performance. The photothermal reproducibility of MPDA-CPT-MnO2 nanocomposites was assessed to be excellent. These photothermal tests indicated that the MnO2 coating displayed no significant effect on the photothermal conversion efficiency of MPDA nanoparticles and the obtained MPDA-CPT-MnO2 nanocomposites owned good photothermal performance.
The coated MnO2 layer was acid-responsive, which can degrade to release O2 in the presence of H2O2. The MnO2 layer was successfully coated onto the surface of nanocomposites that can prevent drug leakage. During this degradation process, Mn2+ was generated and can act as a Fenton-like agent to produce highly toxic •OH. After the Fenton-like reaction, Mn2+ was converted into Mn4+, which tended to proceed redox reaction with GSH to generate Mn2+ and deplete GSH. It was demonstrated that the production of •OH by the formed MPDA-CPT-MnO2 nanocomposites was in a concentration-dependent manner.
4T1 cells were used to assess the performance of MPDA-CPT-MnO2 nanocomposites at the cellular level. The data demonstrated that MPDA-CPT-MnO2 nanocomposites can be effectively internalized by 4T1 cells. The CCK-8 assay was then used to assess the survival rate of 4T1 cells under different treatments. It was found that MPDA nanoparticles displayed good cytocompatibility in the low concentration range (0–200 ppm). Further data demonstrated the high efficacy of collaborative CT/PTT/CDT for tumor cell killing.
The generated Mn2+ can act as a Fenton-like agent to convert H2O2 into •OH. The H2O2 levels in the MPDA-MnO2 and MPDA-CPT-MnO2 groups decreased greatly when compared to the PBS control group. For intracellular ROS detection, the fluorescence signal in the MPDA-CPT-MnO2 nanocomposite group became stronger, indicating the CPT-induced apoptosis-related ROS production. The fluorescence signal in the MPDA-CPT-MnO2 plus laser group was stronger than all other groups, suggesting that the efficiency of ROS production can be enhanced by the photothermal effect. After the Fenton-like reaction, the produced Mn4+ could react with GSH through a redox reaction to produce Mn2+ and deplete GSH. This could disrupt the reducing capacity of tumor cells and benefit the production of ROS. Further cell viability data demonstrated that the formed MPDA-CPT-MnO2 nanocomposites could cause 4T1 cell apoptosis effectively under laser irradiation.
In conclusion, MPDA-CPT-MnO2 nanocomposites were prepared for collaborative CT/PTT/CDT against tumor cells. The formed MPDA-CPT-MnO2 nanocomposites displayed uniform morphology with a hydrodynamic diameter of 270.1 nm. The loading content of CPT was around 21.4%. They displayed good photothermal conversion effect and photothermal stability. In the presence of acid and H2O2, MPDA-CPT-MnO2 nanocomposites degraded to produce O2, release CPT, and generate Mn2+ as the Fenton-like agents. The formed MPDA-CPT-MnO2 nanocomposites can be up-taken by 4T1 cells effectively. They can produce ROS and deplete GSH in 4T1 cells, finally causing cell apoptosis. Cell viability revealed that the developed MPDA-CPT-MnO2 nanocomposites can kill tumor cells with high efficacy through a collaborative strategy.
Abbreviations
•OH: | hydroxyl radicals |
CCK-8: | Cell Counting Kit-8 |
CDT: | chemodynamic therapy |
CLSM: | confocal laser scanning microscopy |
CPT: | camptothecin |
CT: | chemotherapy |
DCFH-DA: | 2’7’-dichlorodihydrofluorescein diacetate |
DI: | deionized |
DTNB: | 5,5’-dithiobis (2-nitrobenzoic acid) |
GSH: | glutathione |
H2O2: | hydrogen peroxide |
KMnO4: | potassium permanganate |
MB: | methylene blue |
MnO2: | manganese dioxide |
MPDA: | mesoporous polydopamine |
NIR: | near-infrared |
PBS: | phosphate buffer saline |
Pluronic® F-127: | poly(ethylene glycol)-block-poly(propylene glycol)-block-poly(ethylene glycol) |
PTT: | photothermal therapy |
ROS: | reactive oxygen species |
TMB: | trimethylbenzene |
UV-Vis: | ultraviolet-visible |
Declarations
Author Contributions
YO: Data curation, Formal Analysis, Writing—original draft. YC: Formal Analysis, Investigation. TX: Formal Analysis, Investigation. YS: Formal Analysis, Investigation. SZ: Formal Analysis, Investigation. CC: Formal Analysis, Investigation. YT: Formal Analysis, Investigation. LH: Formal Analysis, Investigation. HL: Conceptualization, Funding acquisition, Writing—review & editing.
Conflicts of interest
The authors declare that they have no conflicts of interest.
Ethical approval
Not applicable.
Consent to participate
Not applicable.
Consent to publication
Not applicable.
Availability of data and materials
Not applicable.
Funding
This research was funded by the National Natural Science Foundation of China [No. 51703184]. The funders had no role in study design, data collection and analysis, decision to publish, or preparation of the manuscript.
Copyright
© The Author(s) 2023.