Abstract
Self-neuronal regeneration is often limited or nonexistent after neuronal cell damage, making new technologies necessary for treating neurological damage. Although the brain can partially compensate by increasing its plasticity, these compensatory mechanisms can never fully restore the pre-damage state. Analysis of the literature regarding stem cell therapy in case of neurological disorders. Stem cells have shown promise for treating various neurological disorders and disabilities due to their regenerative capacity. Transplanting or administration of different types of stem cells has yielded promising results in animal models and early clinical trials. However, concerns remain regarding their implementation. The type of stem cell used, the optimal method and route of administration, the number of stem cells administered, preconditioning, and the injection schedule all need to be determined. Additionally, the long-term safety of stem cell treatment and the recipient’s age requires further investigation. Despite these concerns, stem cell therapy holds tremendous promise for treating neurological disorders, and continued research and well-designed studies will be crucial for unlocking its full potential.
Keywords
Neural stem cells, embryonic stem cells, induced pluripotent stem cells, adult mesenchymal stem, quantity, route of administration, graphene, neurological disordersIntroduction
Despite its sophisticated nature, the central nervous system remains mysterious and difficult to understand. Many disorders that affect the central nervous system can lead to irreversible impairments and are often associated with cognitive and physical disabilities.
Unfortunately, the adult brain has limited ability to self-regenerate when it is damaged, making it difficult to achieve full recovery. Although there is some potential for endogenous regeneration in the central nervous system, it is not enough to achieve complete recovery. Furthermore, pharmacological therapies that could address the underlying disease processes are rare and often come with inherent drawbacks, such as temporary efficacy, lack of full neurological recovery, or overwhelming cost [1].
Therefore, new therapeutic approaches need to be explored to address brain damage related to central nervous system disorders or diseases. Stem cell therapy has opened new possibilities for treating several neurological disorders. Due to their ability to regenerate and repair, stem cell therapy appears to be a promising candidate for achieving full neurological recovery. Animal studies have shown improvements in neurological function through restorative processes [2]. In stroke and Parkinson’s disease, some recovery has been observed through plasticity and brain remodeling [2]. The mechanism of action of cell therapies is different from drugs. Drugs such as “recombinant tissue plasminogen activator” have a therapeutic role only during a narrow time window and are associated with potential risks, even lethal ones. Stem cell therapies have different mechanisms of action and a wider therapeutic time window [3].
The mechanisms of action, the types, and the properties of stem cells as their potential therapeutic approach in neurological disorders
Overall, stem cells have unique properties that make them valuable in regenerative medicine and the treatment of various diseases and injuries. Their ability to differentiate into multiple cell types, migrate to damaged areas of the body, and secrete growth factors make them a promising tool for repairing and regenerating tissues and organs [4]. Upon transplantation, stem cells can migrate and home toward injured areas of the brain, a process attributed to the expression of growth factors and chemokines [4]. Once at the site of injury, stem cells differentiate into host tissue cells, replacing injured and necrotic neuronal tissue [5]. Through their paracrine mechanisms, stem cells further reduce injury and stimulate endogenous cells to repair and restore the normal functioning of neurons [6]. Additionally, stem cells secrete an array of neuroprotective growth factors that activate several signaling pathways, thereby enhancing differentiation, survival, and maintaining neuronal functions [7]. Also stem cells have angiogenesis, immunomodulatory, and anti-inflammatory paracrine functions by producing growth factors and interleukin [8, 9] (Figure 1).
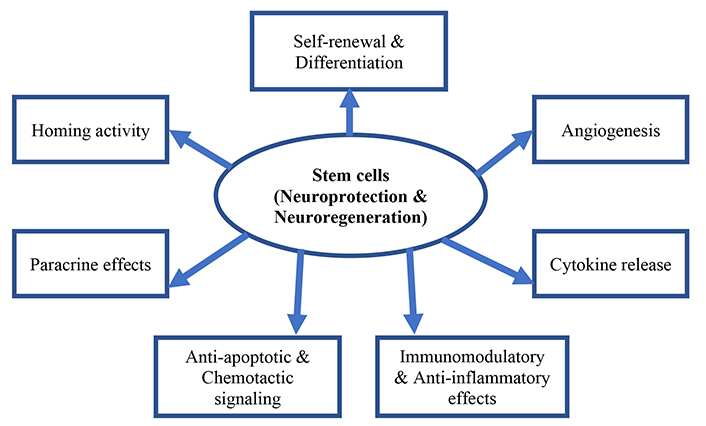
Potential therapeutic actions of stem cells as “neuroregenerative and or neuroprotective” effect in neurological disorders
Note. Adapted from “Stem Cell Therapy in Pediatric Neurological Disabilities” by Sharma A, Sane H, Gokulchandran N, Badhe P, Kulkarni P, Pai S, et al. In: Uner Tan, editor. Physical Disabilities. United Kingdom: IntechOpen; 2017. (http://dx.doi.org/10.5772/67656). CCBY.
The properties mentioned in Figure 1 are crucial when considering stem cell transplantation for brain damage. Stem cells possess the remarkable ability to both self-renew and differentiate into multiple cell lineages [10]. There are various types of stem cells, including neural stem cells (NSCs), embryonic stem cells (ESCs), induced pluripotent stem cells (iPSCs), and adult mesenchymal stem cells (MSCs).
NSCs
Originate primarily from the subventricular zone and sub-granular zone of the hippocampal dentate gyrus. During embryonic development of the central nervous system, NSCs give rise to neurons, astrocytes, and oligodendrocytes [11]. In vitro, self-renewing NSCs can generate these same cell types [12]. Additionally, derived neurons can be supported for prolonged culture using growth factors such as epidermal growth factor [13], fibroblast growth factor-2, and brain-derived neurotrophic factor [14].
These properties enable in vitro-cultured NSCs to undergo self-renewal and differentiation into various types of neurons and glial cells. NSC transplantation therapies are based on these unique properties [11].
ESCs
ESCs are obtained from the inner cell mass of the blastocyst and can differentiate into all three germ layers due to their pluripotent activities. Therefore, they have been the focus of research as a treatment for different neurological diseases. After transplantation, they can differentiate into neurons and glial cells [15] as well as exhibit long-term stability allowing them to integrate the neurological circuitry [16]. By secreting neurotrophic factors, ESCs have a trophic effect on neuronal regeneration at the injured site [17].
Neurons derived from ESCs and possessing appropriate cortical identity have the potential to contribute to the reconstruction of damaged cortical circuitry of the same areal identity [18]. This has been demonstrated in studies using medial ganglionic eminence-like progenitor cells and retinal progenitor cells differentiated from ESCs, where their regenerative potential has shown improvement in learning and memory in Alzheimer’s disease, as well as the structure and function of the retina in retinal degenerative diseases [19, 20].
iPSCs
iPSCs are laboratory-engineered cells that mimic ESCs by converting tissue-specific cells, like skin cells, into pluripotent cells that can give rise to all cell types in the body. While iPSCs share many similarities with ESCs, they are not the same. The successful induction of somatic cells into iPSCs is a significant milestone in stem cell and regeneration research.
Takahashi and Yamanaka [21] first achieved the induction of pluripotent stem cells from mouse embryonic and adult fibroblasts by introducing four factors (Oct3/4, Sox2, c-Myc, and Klf4) under ESC culture conditions. Yu et al. [22] later established another induction method for iPSCs using a combination of Oct4, Sox2, Nanog, and Lin28. iPSCs generated from both methods were similar to ESCs in terms of morphology, proliferation, expression of cell-surface markers, and gene expression profiles.
Takahashi et al. [23] also successfully induced iPSCs from human dermal fibroblasts using the same four factors. These iPSCs were able to generate three germ layers in vitro and in teratomas, demonstrating their potential as a candidate for cell therapy. Other cells, such as hepatocytes, circulating T lymphocytes, and keratinocytes, have also been reprogrammed into iPSCs [24].
The potential uses of iPSCs range from constructing disease models to patient-specific therapeutic transplantations [25]. The availability of iPSCs from patients suffering from a particular neurological disease is already contributing to the development of better disease models. An iPSC-based model for neurodegenerative diseases such as Alzheimer’s disease has been established [26], and iPSC derivatives have been used to investigate the pathogenesis of retinal degenerative diseases [27]. Transplantation of human iPSC derivatives has shown promising results in some neurological disease models, such as spinal cord injury (SCI) [28], intracerebral (IC) hemorrhage [29], and retina-related diseases like macular degeneration [30]. Overall, iPSCs have the potential to revolutionize the field of regenerative medicine and offer new avenues for understanding disease pathogenesis and developing effective therapies.
MSCs
MSCs are pluripotent stem cells especially located in connective tissues, also called mesenchymal stromal cells. First discovered in human bone marrow [31] but can also be isolated from various human tissues such as peripheral blood, adipose tissue, muscle, skin, placenta, and amniotic fluid [32, 33]. Researchers discovered that MSCs can differentiate into cell types of all three germ layers [32]. This makes MSCs a therapeutic source for various neurological diseases. Their neurogenic and immune-modulatory properties make them a suitable cure for different neurological disorders [34]. In fact, autologous bone marrow-derived MSC treatments for Parkinson’s and Alzheimer’s diseases, have shown tremendous therapeutic efficacy [35, 36].
Indeed, MSCs have many advantages: easily accessible; no tumoral formation; easy to cross blood-brain barrier (BBB), and no ethical issue regarding their clinical use. Other advantages also of great importance are the tropism of MCSs not only to attract other MSCs, and to migrate to the cerebral lesion site, but also to activate signaling pathways to promote paracrine secretion of cytokines and growth factors.
MCSs display vasculogenesis and neuroprotective properties making them a suitable candidate for cell transplantation in neurological disorders. Also, due to their immune suppressive capability, no immunological rejection should be expected as well as their ability to be transplanted across the allogenic barrier [37–39].
Despite the advantages mentioned above, the application of stem cell therapy using MSCs remains limited. MSCs have shown a low survival rate, limited engraftment to damaged areas, as well as low differentiation into fully functional tissues [40]. Studies have suggested that their potential beneficial effect is primarily due to their trophic effect rather than the replacement of damaged or lost brain tissue [37, 41]. Additionally, bone marrow MSCs have an anti-inflammatory and immunomodulatory role [38, 41]. However, there is currently no evidence that transplanted MSCs, even if transdifferentiated into neurons, can integrate into the neuronal circuitry of the host nervous system [41].
Stem cell as a therapeutic approach in neurological disorders
Neurodegeneration leads to a gradual deterioration of brain functionality secondary to neuronal and other cell loss of the central nervous system [42]. Stem cells when used as a therapeutic approach could salvage neurons from deterioration and enhance repair of the damaged brain’s circuitry [43]. In this paragraph, different types of stem cells used as a therapy for neurological disorders and diseases will be briefly highlighted, also some promises and concerns will be discussed.
Diseases such as Parkinson’s [44], Huntington’s [45], ischemic stroke [46], traumatic brain injury (TBI) [47], amyotrophic lateral sclerosis [48], Multiple sclerosis [49], Alzheimer’s [50], SCI [51] have been used as a targeted treatment for stem cell therapy.
It is highlighted in Table 1 the use of different types of stem cell therapy, the model used whether animal or clinical studies (only the published clinical studies are reported), the number of stem cells transplanted, the route of administration as well as the potential beneficial effects and the risks.
Preclinical and clinical studies of stem cell transplantation in neurological disorders
Type of stem cells | Neurological disorders | References | Models | Administration’s route | Quantity of stem cells | Effects | Risks |
---|---|---|---|---|---|---|---|
NSCs | Parkinson’s disease | [41, 44] | Rats | IV, IC | 2 × 106 cells | Ameliorated Parkinson dyskinesia and promoted neurogenesis | Risk of cancer development |
Huntington’s disease | [96] | Primate, rats | Transplanted into brain | 5 × 104–1 × 106 cells | Reduction of lesion size and proliferation in the striatum | ||
Stroke | [45] | Mice | Transplanted into brain | 2 × 105 cells | Improved sensorimotor functioning and reduced infarct size | ||
[97] | Human | IC | 2 × 106 cells | It has been shown to be safe and effective in improving NIHSS scale | |||
IV | Escalation dose from 3 × 105–1 × 107 cells | Induces neurogenesis in the human brain | |||||
TBI | [46] | Mice | Transplanted into brain | 2.5 × 105–2 × 107 cells | Early transplant showed an enhanced functional recovery, while a delayed transplantation seems to be more controversial | ||
Amyotrophic lateral sclerosis | [47] | Mice, rats | IV | 2.5 × 105–1 × 106 cells | Increased life span, improvement in motor neuron survival and slow down disease progression | ||
[98] | Human | Transplanted in the spinal cord, transplanted in the motor frontal cortex | 5 × 106–1.6 × 107 cells | Improved the life span and reduced disease progression | |||
Alzheimer’s disease | [99] | Rats, mice | Transplanted into brain | Rats: 1 × 105–1 × 106 cells; mice: 1.5 × 104 cells | Enhanced synaptogenesis and improved learning and memory | ||
ESCs | Parkinson’s disease | [100] | Mice, rats, monkey | IC | Mice: 1.5 × 105 cells; rats: 2.5 × 105 cells; monkey: 7.5 × 106 cells | Neuroprotection and neurogenesis | Tumor formation; ethical issue |
Huntington’s disease | [101] | Mice | Transplanted into brain | 1 × 105 cells | Reduction in cerebral lesion size and proliferation in the striatum | ||
Multiple sclerosis | [101] | Mice, rats | Intraventricular | 1 × 106 cells | Consistent beneficial effect | ||
Human | IV | Not specified | Inconsistent results | ||||
Alzheimer’s disease | [19, 20, 102] | Mice, rats | Transplanted into brain | 1 × 105–1 × 106 cells | Improved learning and memory | ||
iPSCs | Parkinson’s disease | [103] | Mice | Transplanted into brain | 1.5 × 105 cells | Neuroprotective and neurogenesis | Risk of cancer and teratoma formation |
Huntington’s disease | [45, 104, 105] | Rodent | IV, transplanted into brain | 1 × 106 cells | Migration to striatum and differentiation into glial cells | ||
TBI | [56] | Rats | IV | 2 × 106–1 × 107 cells | Long-term improvement in spatial learning and attenuation of neuroinflammation | ||
Amyotrophic lateral sclerosis | [57] | Mice | In culture cells as well as in mice | 1 × 106 cells | Increased life span | ||
Alzheimer’s disease | [26] | Transgenic mice | Transplanted into brain | Not specified | Reduction of brain amyloid beta | ||
MSCs | Parkinson’s disease | [36] | Human | IV | 1 × 106 cells | Exert therapeutic effect by protecting dopaminergic neurons and maintaining the nigrostriatal pathway | Short survival time of the cells; homing issues |
[106] | Mice | Transplanted into brain | Not specified | Subjective improvement of the gait and the facial symptoms | |||
Stroke | [66] | Mice, rats | IC, IV | 1 × 108 cells | Improved sensorimotor functioning and reduced infarct size | ||
[67] | Human | IV | |||||
TBI | [107] | Rats | Transplanted into brain | 3 × 105–4 × 106 cells | Reduced inflammatory cytokines, reactive astrogliosis, and edema | ||
Amyotrophic lateral sclerosis | [38, 108] | Human, mice | IV | 1.7 × 107 cells | Positive safety outcome and increased life span | ||
Multiple sclerosis | [48] | Mice, rats | Transplanted into brain | 2 × 107 cells | Alleviated symptoms and positive effect on cytokines | ||
[38] | Human | IV | 2.5 × 106 cells | Safe and improvement of symptoms | |||
Alzheimer’s disease | [35] | Mice | Intracerebroventricular, IC, IV | Not specified | Improved cognitive function and reduced amyloid beta | ||
SCI | [64] | Rats | IC, IV | Not specified | Improve motor recovery | ||
[51] | Human | IV | 8 × 105–4 × 108 cells | Improve sensory and motor score |
IV: intravenous; NIHSS: National Institutes of Health Stroke Scale
NSCs obtained from fetal brain tissue can differentiate into multiple cell types, but they are primarily committed to the neuronal lineage. Unfortunately, these cells are both difficult to obtain and often present in limited quantities [52].
Administration of NSCs in animal models improved dyskinesia in Parkinson’s, reduced lesions in Huntington’s disease, reduced the lesion size in stroke and TBI, enhanced synaptogenesis, and improved learning memory in Alzheimer’s disease, and increased life span in amyotrophic lateral sclerosis. In human small studies, the use of NSCs seemed to be safe (Table 1).
ESCs are pluripotent in nature and could restore brain injuries and neurodegeneration, however, tumor formation restricts their widespread application [37].
In the preclinical setting, ESCs displayed neuroprotection and neurogenesis in Parkinson’s disease, reduced cerebral lesions in Huntington’s disease, and improved learning and memory. However, in a clinical setting, the results seemed inconsistent (Table 1).
iPSCs have become a widely available resource for research as various iPSC cell lines are being produced in bulk and are commercially available. The use of poly(ADP-ribose) polymerase-1 (PARP-1) to produce iPSCs has been shown to be effective in reducing the risk of tumor formation [53]. However, the teratoma formation risk has not been completely eliminated.
iPSC can be derived from various somatic cells of the body, including peripheral blood cells, hepatocytes, stomach cells, and keratinocytes [54]. Interestingly, even a small blood quantity as low as just 10 μL drawn from fingertips can be used to generate iPSCs [55].
Patient-derived iPSCs are widely used for modeling various human neurodegenerative diseases [56, 57]. For instance, spinal muscular atrophy has been efficiently modeled in vitro using patient-derived iPSCs, which accurately depict the disease phenotypes [58]. However, iPSCs do have a few limitations. Neurodegenerative disorders are generally late-onset diseases, and their symptoms begin to manifest with increasing age. Thus, modeling such diseases via animal models is not only time-consuming but also costly. However, when somatic cells are reprogrammed back into an embryonic-pluripotent-like state, which enables them to develop into any human cell, they lose the age-associated features [59]. During this transformation process, iPSCs undergo rejuvenation, and their embryonic pluripotent state is re-established. It has been reported that even aged donor-derived iPSCs undergo rejuvenation, displaying a loss in markers of senescence, enhanced mitochondrial fitness, and increased telomere length [59]. However, patient-derived iPSCs still lack the age-related phenotypes necessary for effectively modeling late-onset neurodegenerative diseases. Nevertheless, this hurdle has been largely overcome by progerin-induced aging in iPCSs [60].
Studies in rodents have displayed an improvement in memory, neuroprotection, and increased lifespan in Alzheimer’s, Parkinson’s, and amyotrophic lateral sclerosis diseases (Table 1).
MSCs multipotent cells also find widespread application because they are immunomodulating in nature. These immunomodulating characteristics allow them to escape the host’s immune system surveillance and hence successful transplants without the use of immunosuppressant drugs and avoiding immunosuppressant drug side effects [61].
Other studies have shown that MSCs may be beneficial in SCI through direct contact with immune cells or paracrine-release signaling molecules to reduce the inflammatory response at the level of the injured spinal cord. Also, MSCs release neurotrophic factors such as BDNF and b-NGF to promote axon regeneration. Furthermore, MSCs regulate signaling pathways to inhibit glial scarring. Hence, mitigation of glial scarring facilitates axon regeneration and herby improves the sensory and motor scores [62].
In preclinical studies, the use of stem cell therapy improved sensorimotor functioning, reduced inflammatory cytokines, alleviated symptoms, and improved cognitive function in stroke, SCI, TBI, multiple sclerosis, and Alzheimer’s disease respectively (Table 1).
Despite a number of animal experiments, the data generated fails to be translated into human diseases [63]. The lack of translational implementation could be explained by several issues: the quality of the studies, the type of the animal model, the therapeutic modalities, timing, preconditioning, etc. [51, 63, 64].
Study quality and the animal model
The considerable degree of heterogeneity of the disease phenotype in clinical settings when compared to homogeneity in animal studies, is an affirmation of the lack of standardization (Table 1). The non-confirmation of the positive preclinical studies in humans could partially be explained by the limited number of patients included and the heterogeneity of the studied population [63]. Also, a lack of blinding because of ethical considerations [63]. Equally important is the choice of the animal model as a therapeutic target. Most studies were using rodents as an animal model [65]. We should question whether rodents could be a good model or even any animal model would be appropriate to mimic the clinical setting. Even using a high phylogenic species such as nonhuman primates remains an issue. These animal models require laborious post-operative care, are a homogenous population in contrast to humans, and do not have underlying co-morbidities.
Several steps have been taken to standardize preclinical studies, as proposed in the Stem Cell Therapy as Emerging Paradigm in Stroke (STEPS) initiative [66, 67]. However, some researchers argue that certain issues related to the translation of stem cell therapy to human patients may be better addressed through careful studies in human models, rather than additional preclinical studies [65].
Cell type
When transplanting stem cells, the choice between autologous and allogeneic stem cell transplantation should be carefully considered, considering the risks and benefits for each patient. The use of allogenic stem cells implies the risk for immune reactions and graft-versus-host disease which automatically require immunosuppressants. The choice of autologous stem cell transplantation eliminates the risk of immune reactions and graft-versus-host disease, but it may not be possible for all patients.
Manufacturing steps for stem cell transplantation, such as genetic modification, cell expansion, and reprogramming (using viral integration) should be carefully controlled to minimize the risk of tumor formation [68]. This requires stringent quality control measures and adherence to good manufacturing practices. The time and requirements for cell expansion may differ between cell types and medicinal manipulations, which may affect the availability and cost of stem cell therapies. These factors should be carefully considered when developing and implementing stem cell therapies [69, 70].
Dose of stem cells administered
When evaluating animal studies, often a higher cell concentration was used (ranging from 5 × 106 to 5 × 107) [71–73]. Others proposed 2 different administrations rather than one single administration [74]. But here, it seems that the benefit might reside in the absolute higher dose caused by the double injection, rather than the number of injections itself.
According to the literature, a single high concentration of 3 × 106 cells is considered better than multiple low-dose injections at different times [75]. The cell concentration-response curve probably follows a U-shape rather than a linear progression, with a higher number of cells leading to a ceiling effect. One study described a severe ipsilateral eye inflammation followed by acute mortality of the studied animals after administration of 107 cells.
Besides the cell concentration, the type and the size of cells are of great importance. MCSs have the tendency to aggregate in multicellular globules, thus a higher concentration could result in vascular embolization. One study suggested that 5 × 106 cells as the maximum number of cells that could be safely transplanted [76]. Others concurred with this statement, where stem cell injections as high as 5 × 107 cells caused high mortality rates due to embolisms [71]. Based on previous publications, it seems that a cell quantity of 5 × 106 cells is an ideal cell quantity number [71]. Another study showed that animals died when they received greater than 3 × 107 cells, stating 1 × 107–2 × 107 cells might be the optimal range [77]. Reducing the number of cells would not be effective since a lower number of cells would not be enough to enter the brain for effective treatment [65, 72, 73]. Besides the number of cells, others have proposed that cell size and velocity of injection are of equal importance [70]. Slower injection might diminish sludging and thereby allow higher cell doses [70].
In clinical trials, different cell doses have been used. Based on mean body mass, Bang et al. [78] proposed that 1 × 108 cells/patient is the human dose equivalent to the dose used in rodent stroke models. They considered 1 × 105–3 × 106 cells/rat as the effective dose used in rodents. Two other trials proposed different doses [73, 79]. Other studies have shown that the dose of stem cells and more particularly MSC at a dose between 8 × 105 and 4 × 108 administered intravenously or in the subarachnoid space improve the neurological function in 33.3% of patients with the acute phase of SCI while in the chronic phase of SCI did not result in a significant improvement of the outcomes [80]. The absence of improvement in the chronic SCI patient could be explained by the presence of glial scarring which physically blocks axon regeneration [81].
Researchers must carefully consider the potential risks and benefits of different dosing strategies to ensure safe and effective stem cell transplantation. Research is needed to determine the optimal dose for different conditions and types of stem cells (Table 1).
Way of administration
Preclinical studies use mainly three different injection routes: IC, intra-arterial (IA), and IV. IC stem cell transplantation seems to be the most effective route and results in the highest number of cell deposits into the target area of the brain (Table 1). However, this is also the most invasive route and studies have shown that the stereotactic inoculation of cells can cause hemorrhage or injury (probably by needle insertion), fluid loading, neuronal cell deaths, reactive gliosis, and micro-calcification [77, 82].
Both intravascular routes can avoid unnecessary brain damage compared to the invasiveness of the IC procedure. IA administration route has been correlated with a decrease in infarct volumes, functional recovery, and a high number of surviving stem cells. IA injection, however, holds the risk of cerebral embolisms and reduction of blood flow when high doses of stem cells are infused [70, 82]. IV injection is the less-invasive, simplest, and practical route of administration, but has the disadvantage of dispersing cells throughout the body, resulting in fewer cells reaching the brain parenchyma because of entrapment in the lungs and other peripheral organs [70, 71, 82].
Lately, tissue engineering created a new perspective and shown a potential solution to the dismal poor administration and regenerative capabilities of neuronal systems in re-establishing axonal connections after injury of diseases.
Graphene scaffolds, three-dimensional graphene foam, and other biomaterials can serve as substrates for the culture and differentiation of NSCs. NSCs grown on these materials can be guided to differentiate into specific neural cell types, such as neurons and glial cells. This approach can be utilized to generate neural tissue for transplantation, potentially replacing damaged or lost cells in neurological disorders [83].
Graphene-based materials (layers of carbon atoms arranged in a two-dimensional honeycomb lattice) possess unique properties that can facilitate improved cellular interactions. For example, graphene’s high surface area and electrical conductivity can enhance cell adhesion, proliferation, and differentiation. By providing a favorable environment for stem cells to grow and develop. Graphene scaffolds and foam can potentially support the regeneration of neural tissue.
Graphene’s electrical properties also enable the integration of electrical stimulation into stem cell therapy for neurological disorders. Electrical signals applied through graphene-based scaffolds can promote neural cell differentiation, guide axonal growth, and enhance neural network formation. This electrical stimulation can potentially aid in the functional recovery of damaged neural circuits [84, 85].
It is important to note that the potential of graphene-based materials in neurological stem cell therapy offers exciting prospects for the advancement of progressive nanotechnology and generative stem cell therapy. There are still challenges to overcome. These include optimizing the biocompatibility, long-term stability, and integration of graphene materials with neural tissue. Further research and development are necessary to fully understand their interactions with cells and their long-term effects in vivo [83].
Timing of stem cell administration
Several studies have compared different administration times. For example, the study of Komatsu et al. [86] delivered stem cells in 3 time periods from 7 days after middle cerebral artery occlusion (MCAo) until 28 days after MCAo. The stem cells injected at 7 days post-MCAo did significantly better than the cells transplanted at 28 days [86]. Administration at 4 weeks post-MCAo might thus be too late to be effective [87]. The study of Nam et al. [79] on the other hand, compared 1 h, 1 day, or 3 days post-MCAo injection. The 1 h post-MCAo transplanted group showed maximum neurological recovery, in both functional and structural ways [78].
Several other preclinical studies support the early administration of stem cells. It seems that 24 h is the most optimal administration time because this might go along with the time of opening of the BBB [70, 82, 88]. However, this early administration has some practical implications when implemented in a real clinic setting. The preparation of autologous stem cells needs time for production and expansion before administration which will delay the administration. During the acute phase of the neurological disability, patient stabilization is mandatory to reduce secondary brain damage. All these would not make the patient suitable for early administration. Pilot studies have shown that many patients undergo marked improvement or worsening in the first week after stroke or trauma [89, 90]. In both cases, early transplantation of stem cells would be unethical, and late transplantation could be inefficient [63]. The exact optimal timing remains to be defined.
Preconditioning for improved stem cell survival after transplantation
Despite the significant advancements in stem cell therapy, various factors hinder the acceptance and survival of stem cells. Within hours of transplantation, a considerable number of donor cells die, which is attributed to pathological processes, including local immunological and inflammatory responses, loss of trophic factors, and reduced perfusion and nutrients due to local primary factors responsible for the initial ischemic insult [91]. The efficacy of stem cell transplantation depends on the number of cells retained and active at the graft site, although increasing the amount of cell engraftment may overcome massive cell death and washout. Still, transplanting a such large number of cells could induce aggregation, vascular embolism, and inflammation generated by graft cell death and hence jeopardize the potential beneficial effect [71, 76, 77]. Therefore, it is essential to reinforce donor cells to withstand the microenvironmental rigors of the infarcted site by optimizing cell engraftment without increasing complications [92]. Previous study has shown that priming cells before transplantation with cytokines to a state of “readiness” by stimulating their survival pathways can fortify cell engraftment, leading to enhanced survival and overcoming numerous problems during the post-engraftment period [93].
Tissue engineering such as graphene scaffolds and three-dimensional graphene foam hold great promise, further research and development are necessary to fully understand their interactions with cells and their long-term effects in vivo. However, these materials offer exciting prospects for the advancement of progressive nanotechnology and generative stem cell therapy [83–85].
Additionally, the age of the recipient is a crucial factor, with young patients expected to have a greater potential for neuroplasticity and anti-inflammatory effects. Most neurological disorders however are expected to increase with age. Still, other neurological disorders such as TBI and neonatal stroke occur primarily in a young population. The anti-inflammatory effect of stem cell therapy is thus reasonably expected to produce greater neurological rescue from inflammation induced in young brains compared to adults. This was observed in the rat model of TBI [94] as well as in the hypoxemic ischemic neonatal mice model [95].
Conclusions
Stem cell therapy has shown promising results in treating various neurological disorders in animal models. This animal approach allows a better understanding of the mechanisms involved as well as a better comprehension of the healing processes after a neurological disorder. Still translating these results to human clinical trials requires a significant amount of research and well-designed studies. High-quality preclinical studies should address factors such as the optimal cell type, quantity of stem cells administered, way of administration, underlying co-morbidities, and timing of delivery. These factors can significantly impact the effectiveness of stem cell therapy and must be carefully controlled and tested in preclinical studies to ensure successful translation to clinical settings. Additionally, it is crucial to ensure that preclinical studies are large and well-designed to provide robust evidence for the safety and efficacy of stem cell therapy. Despite the challenges, stem cell therapy holds tremendous promise for the treatment of neurological disorders. With continued research well-designed studies and implementation of tissue engineering, we may one day be able to unlock the full potential of this groundbreaking technology.
Abbreviations
ESCs: |
embryonic stem cells |
IA: |
intra-arterial |
IC: |
intracerebral |
iPSCs: |
induced pluripotent stem cells |
IV: |
intravenous |
MCAo: |
middle cerebral artery occlusion |
MSCs: |
adult mesenchymal stem cells |
NSCs: |
neural stem cells |
SCI: |
spinal cord injury |
TBI: |
traumatic brain injury |
Declarations
Author contributions
SHI: Conceptualization, Investigation, Writing—original draft, Writing—review & editing, Validation.
Conflicts of interest
The author declares that he has no conflicts of interest.
Ethical approval
Not applicable.
Consent to participate
Not applicable.
Consent to publication
Not applicable.
Availability of data and materials
Not applicable.
Funding
Not applicable.
Copyright
© The Author(s) 2023.