Abstract
Aim:
To investigate alterations in transcription of genes, encoding Ca2+ toolkit proteins, in oesophageal adenocarcinoma (OAC) and to assess associations between gene expression, tumor grade, nodal-metastatic stage, and patient survival.
Methods:
The expression of 275 transcripts, encoding components of the Ca2+ toolkit, was analyzed in two OAC datasets: the Cancer Genome Atlas [via the University of Alabama Cancer (UALCAN) portal] and the oesophageal-cancer, clinical, and molecular stratification [Oesophageal Cancer Clinical and Molecular Stratification (OCCAMS)] dataset. Effects of differential expression of these genes on patient survival were determined using Kaplan-Meier log-rank tests. OAC grade- and metastatic-stage status was investigated for a subset of genes. Adjustment for the multiplicity of testing was made throughout.
Results:
Of the 275 Ca2+-toolkit genes analyzed, 75 displayed consistent changes in expression between OAC and normal tissue in both datasets. The channel-encoding genes, N-methyl-D-aspartate receptor 2D (GRIN2D), transient receptor potential (TRP) ion channel classical or canonical 4 (TRPC4), and TRP ion channel melastatin 2 (TRPM2) demonstrated the greatest increase in expression in OAC in both datasets. Nine genes were consistently upregulated in both datasets and were also associated with improved survival outcomes. The 6 top-ranking genes for the weighted significance of altered expression and survival outcomes were selected for further analysis: voltage-gated Ca2+ channel subunit α 1D (CACNA1D), voltage-gated Ca2+ channel auxiliary subunit α2 δ4 (CACNA2D4), junctophilin 1 (JPH1), acid-sensing ion channel 4 (ACCN4), TRPM5, and secretory pathway Ca2+ ATPase 2 (ATP2C2). CACNA1D, JPH1, and ATP2C2 were also upregulated in advanced OAC tumor grades and nodal-metastatic stages in both datasets.
Conclusions:
This study has unveiled alterations of the Ca2+ toolkit in OAC, compared to normal tissue. Such Ca2+ signalling findings are consistent with those from studies on other cancers. Genes that were consistently upregulated in both datasets might represent useful markers for patient diagnosis. Genes that were consistently upregulated, and which were associated with improved survival, might be useful markers for patient outcome. These survival-associated genes may also represent targets for the development of novel chemotherapeutic agents.
Keywords
Oesophageal adenocarcinoma, Ca2+ toolkit, acid-sensing, voltage-gated Ca2+ channel subunits, junctophilin 1, acid-sensing ion channel 4, transient receptor potential ion channel melastatin 5, secretory pathway Ca2+ ATPase 2Introduction
Globally, oesophageal malignancies are the sixth-leading cause of cancer-related mortality [1]. The age-standardized 5-year net survival for oesophageal cancers (OCs, 2010–2014) was 21.9% for Ireland [2], 16.2% for the UK [2], and 20% for the United States [3]. Causes of poor prognosis include late diagnosis, incomplete resection of tumors, and resistance to chemotherapeutic and radio-therapeutic interventions [4]. Histologically, there are two major distinct forms of OC: oesophageal squamous cell carcinoma (OSCC), derived from epithelial cells, and oesophageal adenocarcinoma (OAC), arising from glandular cells [5]. OSCC is most prevalent in Southeast Africa and Asia [5]. By contrast, OAC is the most prevalent form in North America and Europe [5]. Risk factors for OAC include obesity, Barrett’s esophagus (BO, the replacement of normal, squamous epithelia with metaplastic columnar epithelia [6]), and gastroesophageal reflux disease (GORD) [1–7]. Two stomach-derived stimuli impacting oesophageal cells, because of GORD, are hydrochloric acid and bile acids (BAs) [8–9]. Little is known, however, about how oesophageal cells detect and respond to these stimuli [10].
Intracellular calcium (Ca2+) is a key second messenger in the cell [11–13]. In response to both extracellular and intracellular cues, cytoplasmic free Ca2+ [Ca2+]c can be increased by up to two orders of magnitude [14, 15]. Such [Ca2+ ]c transients regulate almost every aspect of cell biology including cell motility, gene expression, and cell death [11–15]. These increases in [Ca2+]c can occur through several different mechanisms [15, 16]. [Ca2+]c can be increased through the gating of ion-channel proteins, located in the plasma membrane (PM) or intracellular organelles, allowing the influx or release of Ca2+ into the cytoplasm [11]. These channels include those gated by changes in membrane potential [voltage-gated Ca2+ channels (VGCCs)], by ligands, by second messengers, by multiple stimuli [such as transient receptor potential (TRP) channels] or by the depletion of intracellular Ca2+ stores [store-operated Ca2+-entry (SOCE) channels, including Orai channels, which are gated by interactions with stromal interaction molecule (STIM) proteins] [11, 14, 15, 17]. Intracellular Ca2+ stores, such as the endoplasmic reticulum (ER) and sarcoplasmic reticulum (SR), act as reservoirs of Ca2+; this Ca2+ is released via channels such as the inositol 1,4,5-trisphophate (IP3) receptors (IP3Rs) and ryanodine receptors (RyRs) [11, 14, 15, 17]. Golgi, secretory pathway Ca2+ ATPase (SPCA) 1 and 2, and the SR/ER Ca2+-ATPases (SERCAs 1–3) are pumps that actively accumulate Ca2+ into intracellular stores, thereby decreasing [Ca2+]c [11, 14, 15, 17]. [Ca2+]c is also buffered by mitochondria, whose metabolic activities and effects on cell death are influenced by this second messenger [18]. As such, Ca2+ signalling can be influenced by mitochondrial proteins like those comprising the permeability transition pore, the mitochondrial Ca2+ uniporter (MCU), and mitochondrial Ca2+ exchangers [19, 20]. Changes in [Ca2+]c are detected by effector proteins, including Ca2+-sensors, enzymes, transcription factors, and motor proteins [12, 13]. Together, the Ca2+-regulating and -sensing proteins within a cell can be considered a “toolkit”, which orchestrates stimulus-response coupling, Figure 1.
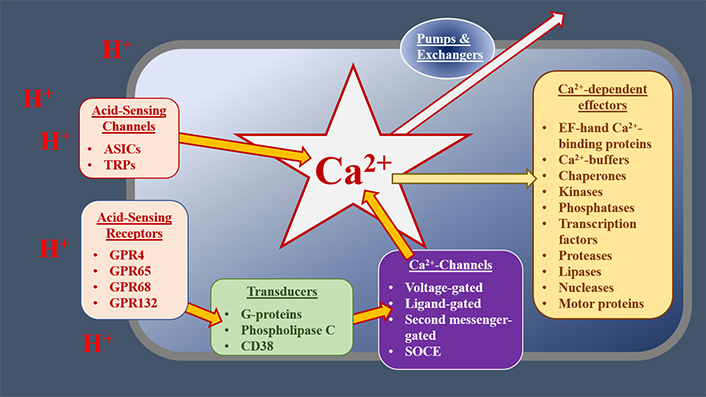
The potential role of the Ca2+ toolkit in OAC cells. At the cell surface, extracellular cues, such as decreases in extracellular pH (increased [H+]), are detected by receptors or cation channels, which gate to allow Ca2+ influx. GPRs activate transducers, such as Gαq/11, which stimulate phospholipase C (PLC) and subsequently increase the concentration of IP3. This increased IP3 concentration, in turn, gates IP3Rs, releasing Ca2+ from the ER. Other Ca2+ channels and receptors respond to distinct stimuli or modify the signals generated by acid-sensing mechanisms. Elevated [Ca2+]c alters the activities of various downstream effectors, resulting in the modification of cell physiology. Normal [Ca2+]c is restored by buffers, pumps, and exchangers, operating in distinct subcellular compartments. Together, these receptors, Ca2+ channels, transducers, transporters, and effectors act as a Ca2+ toolkit, the components of which are distinctive for the type and condition of cells. ASICs: acid-sensing ion channels; GPR: G-protein coupled receptors; CD38: cluster of differentiation 38
Altered Ca2+ signalling can result in pathological or pre-pathological states [12–17]. In cancer cells, the Ca2+ toolkit is remodeled to enhance the hallmarks of malignancy. This remodeling can impact proliferation, metastasis, or the evasion of apoptotic cell death [21]. Remodeled mitochondrial proteins, in particular, play a role in cancer cell proliferation and the evasion of cell death [22–27]. Ca2+ signalling has been shown to play critical roles in the biology of both normal and malignant oesophageal cells [21]. Extracellular Ca2+, for example, promotes the proliferation of human esophageal epithelial cell line (HET-1A) cells [28]. Alteration of the Ca2+ toolkit has been reported to contribute to the tumorigenic phenotype of other cancers, including OSCC [15, 17, 29–41]. Most studies to date have focused on the Ca2+ channel, TRP vanilloid 6 (TRPV6) [17, 34–40]. This channel was reported to be upregulated in breast, colon, ovary, prostate, and thyroid carcinomas [36]. In OSCC, however, TRPV6 was reported to be downregulated [35]. Increased TRPV6-expression has been associated with a better prognosis in cervical carcinoma [37]. The opposite effect (worsened survival with increased expression) has been reported in pancreatic cancer [38] and high-grade prostate cancer [34]. A sex-specific effect was noted in OSCC: male patients with TRPV6-downregulation had a poor 3-year disease-specific survival, whereas female counterparts showed enhanced survival [35]. Several TRPV6-related mechanisms have been proposed. In vitro analysis showed that TRPV6-silencing reduced breast cancer cell proliferation and promoted apoptosis [39]. In prostate-adenocarcinoma cells, TRPV6 supported high proliferation rates by providing constitutive Ca2+-influx for subsequent downstream activation of the nuclear factor of activated T cells (NFAT) [40]. TRPV6-expression is promoted by the activation of vitamin D3, estrogen and androgen receptors [36]. Such an interaction explains, at least in part, the effect of TRPV6 on the hormone-related cancers of the breast and prostate, as well as the sex-specific association of TRPV6 with OSCC survival [36]. Finally, the Orai3 protein works in conjunction with TRPV6 to promote proliferation in prostate and breast cancer [17]. Some study has been carried out on the TRPV2 channel, a channel that belongs to the same family as TRPV6. Patients with OSCC, gastric cancer, triple-negative, breast cancer, and bladder cancer, who had high TRPV2-expression, displayed poorer survival [29–31, 41]. Similar to TRPV6 and TRPV2 (poor prognosis with high expression in numerous cancers), high expression of the PM Ca2+ ATPase 2 (PMCA2) conferred resistance to apoptosis and was associated with a poor prognosis [32]. Other Ca2+-toolkit proteins implicated in various cancers include calcineurin, SERCA pumps, SPCAs, PMCAs, the IP3R, RyRs, STIM proteins, T-Type VGCCs, TRPC1, TRPC3, TRPC6, TRP ion channel melastatin 2 (TRPM2), TRPM7 and TRPM8 [15, 17, 34]. Some Ca2+-permeable channels have been implicated in the enhanced migration of various cancers: TRPC1, TRPM7, TRPM8, TRPV1, TRPV2, TRPV6, STIM1, Ca2+-release activated Ca2+ modulator 1 (Orai1) and some types of VGCCs [17, 33]. It is not known whether there are Ca2+-toolkit changes in OAC and if there is any association with tumor progression or patient survival.
Acidic conditions, occurring during GORD, are a key stimulus for the development of BO [6]; those with BO are 40–50 times more likely to develop OAC [42]. Decreased extracellular pH has been implicated in the proliferation of some cancer-derived cell lines (prostate, colon, lung, and breast cancers; pH ranged from 6.0–6.8) [43–48]. Extracellular acid (EA) has also been implicated in cancer metastasis in other cell lines (prostate, lung, and murine melanoma cancers; pH ranged from 5.9–6.8) [49–51]. Whether acidic extracellular environments result in oncogenesis in OAC, via the development of BO or by other mechanisms, remains unclear. Proposed oncogenic mechanisms include the production of reactive oxygen species (ROS), increased genomic instability, increased proliferation, dysregulation of apoptosis, and increased inflammation [43–56]. Exposure to EA has been linked to ROS production in BO [56]. Roesly et al. [52] showed that chronic exposure to BAs increased genomic instability and proliferation in BO and OAC cell lines. Indeed, the BA receptor farnesoid X receptor (FXR) is significantly overexpressed in BO (compared to normal mucosa, oesophagitis, and OAC) and may contribute to the regulation of apoptosis [53]. In nasopharyngeal carcinoma, BA-induced apoptosis (mediated by caspase-activated deoxyribonuclease) contributed to chromosomal rearrangements [54]. Inflammatory mediators [specifically: ROS, interleukin-1 (IL-1), IL-6, IL-8, and transforming growth factor-beta (TGF-β)], located in the oesophageal mucosa in GORD patients, have also been implicated in carcinogenesis [55]. Oxidative stress, caused by ROS, leads to DNA damage, RNA damage, activation of oncogenes, and inhibition of tumor-suppressor proteins [55]. IL-1, IL-6, and IL-8 enhance epithelial turnover (OAC is epithelia-derived cancer) [55]. TGF-β is generally anti-inflammatory [55]. TGF-β-responsiveness is reduced in OAC due to alterations in its signalling pathway [55]. Altered Ca2+ signalling could be contributing to all of these candidate mechanisms [57–69].
Multiple Ca2+-toolkit-related mechanisms link increased extracellular [H+] to increases in [Ca2+]c and consequent changes in cell physiology [10, 12]. These include TRP channels [TRPA1, TRP ion channel classical or canonical 4 (TRPC4), TRPM2, TRPM5, TRPV1, TRPV4 and TRPV5]; GPRs, linked to PLC-activation and Ca2+-release via IP3Rs (GPR4, GPR65, GPR68 and GPR132); ASICs 1a, 1b, 2a and 3; vacuolar ATPases [ATPase H+ transporting V0 subunit A1 (ATP6V0A1), ATP6V0A2, ATP6V0A4, ATP6V0B and ATP6V0C]; proton exchangers [encoded by Solute Carrier 9A (SLC9A)1-9]; solute-carrier family 4 member A (SLC4A; SLC4A1-5 and SLC4A7-11); chloride-bicarbonate exchangers [solute-carrier family 26 member (SLC26; SLC26A and SLC26A1-10)]; carbonic anhydrases (CA; CA1-4 and CA6-14); and the hydrogen voltage-gated channel 1 (HVCN1) [10, 12, 70–78]. A broad overview of the role of some of these Ca2+-toolkit proteins in the cell has been detailed in Figure 1. Despite the potential impact of these acid-sensing proteins, their roles in the etiology of BO and OAC are currently unknown.
A limited number of studies have evaluated alterations of the Ca2+ toolkit, and their potential association with patient outcome, in OAC. Even less research has investigated how certain Ca2+-toolkit proteins may sense acidic environments and might, as a result, be remodeled to favor carcinogenesis. EA-exposure increased Ca2+-levels in an OAC cell line (pH 5) and in a murine, metastatic, melanoma cell line (pH 5.4-6.5) [60, 61]. Li and Cao [60] showed that the EA-stimulated increase in [Ca2+]c in their OAC cell line activated the nicotinamide adenine dinucleotide phosphate (NADPH) oxidase 5-S enzyme, which subsequently elevated ROS and caused DNA damage.
In the current study, we examined the transcript levels of various components of the Ca2+ toolkit in OAC samples and in normal oesophageal samples, using data from both The Cancer Genome Atlas (TCGA) and the Oesophageal Cancer Clinical and Molecular Stratification (OCCAMS) consortium [79, 80]. We focused on a gene list of Ca2+-toolkit components, updated from an original review of these [13], with the addition of known components involved in acid-sensing [10] and those related to mitochondrial function [19, 20, 22–27].
Specifically, we aimed to:
Interrogate two distinct transcriptomic datasets to assess whether there is differential expression of any of 275 Ca2+-toolkit genes between OAC and normal tissue.
Assess whether there is an association between the expression of Ca2+-toolkit genes and patient survival in OAC.
Examine whether these survival-associated genes are associated with tumor grade or metastasis.
Materials and methods
Two cancer transcriptome datasets were used in this study: TCGA (esophageal-carcinoma subset), accessed via the UALCAN portal [79, 81], and the OCCAMS dataset [80]. The TCGA dataset compared data from 89 OAC-tumor samples with 11 same-patient normal-adjacent tissue (NAT) samples. The OCCAMS dataset compared data from 213 OAC samples with data from 15 normal-tissue samples (from independent OAC cases).
The complete list of genes examined, and their associated proteins are detailed in Table S1. The expression levels of each of these 275 genes were assessed in OAC-tumor tissue and compared to expression levels in normal tissue. The mRNA expression levels of each gene were plotted as heat maps, using a log2 [transcripts per million (TPM) + 1] scaled look-up-table. To determine which genes were most consistently and significantly altered between normal and tumor tissue across both datasets, a dataset-specific weighted rank (the probability of altered expression of each gene, compared to normal tissue, relative to other differentially-expressed genes) was calculated for each gene and the average of both dataset-specific ranks was then taken. The association of each gene with patient survival was investigated using Kaplan-Meier survival analysis. This analysis was carried out initially on all genes in the OCCAMS dataset only; any genes with a statistically significant effect on survival were subsequently analyzed in UALCAN. A comparison was made between patients with high expression of the gene of interest (TPM reads above the upper quartile) and those with low expression (TPM below the lower quartile). To determine which survival-associated genes were selected for further analysis, the extent to which they were differentially expressed across both datasets was evaluated. Again, an average of the two dataset-specific weighted ranks was computed for each gene. Genes having an association with survival, which also had the smallest, average, weighted ranks (1 being the smallest and 75 being the largest) for differential expression, were selected for further analysis.
Selected genes were also examined for differential expression across OAC tumor grades and nodal metastatic stages. Tumour grade refers to changes in the morphology of cells, as assessed by microscopy [82]. Histological tumor grades in this analysis were stratified based on cellular differentiation: Grade 1 was well-differentiated; Grade 2 was moderately differentiated, and Grade 3 was poorly differentiated. The metastatic stage refers to tumor location and whether there was any metastasis in the lymph nodes or in distant sites [82]. For the metastatic-stage boxplots, the following categories were used: N0 corresponded to the tissue having no regional lymph-node metastasis; N1 corresponded to the tissue having metastasis in 1 to 3 axillary lymph nodes; N2 corresponded to the tissue having metastasis in 4 to 9 axillary lymph nodes, and N3 corresponded to the tissue having metastasis in 10 or more axillary lymph nodes.
Statistical analysis
Gene-expression data for tumor versus normal tissue were compared using Welch’s t-tests. Associations of transcript-level (upper quartile versus lower quartile) with patient survival were presented in Kaplan-Meier plots and were statistically compared using log-rank tests. Associations between transcript levels and differentiation grade or lymph-node metastasis were presented as boxplots, compared by analysis of variance (ANOVA) with Tukey post hoc tests. For statistical comparisons, P-values of less than 0.05 were considered significant. An adjustment for multiplicity of testing (MOT) was made (for both UALCAN and OCCAMS data) using the false discovery rate (FDR) method [83, 84], for both the Welch’s t-tests and Kaplan-Meier, log-rank tests. Tukey, honest-significant-differences, post hoc tests served as an appropriate adjustment for MOT for UALCAN and OCCAMS ANOVA tests.
Results
Transcript levels of 275 genes, encoding components of the Ca2+ toolkit, were investigated in two OAC datasets. A total of 392 statistical tests (Welch’s t-tests, Kaplan-Meier, log-rank tests and ANOVA with Tukey post hoc tests) were carried out in the UALCAN portal and 638 in the OCCAMS dataset [data was not available for four genes in OCCAMS: G protein subunit gamma 7 (GNG7), mucolipin 1 (MCOLN1), JPH3, and sorcin (SRI)]. Initial survival analysis of 271 genes was carried out in the OCCAMS dataset (the dataset with the most statistical power). The resulting survival-associated genes significantly associated with survival were then analyzed in UALCAN. One hundred and forty-eight gene variables [gene variable being defined as “a gene and an associated test”, such as “voltage-gated Ca2+ channel auxiliary subunit α2 δ4 (CACNA2D4)-heat map” and “acid-sensing ion channel 4 (ACCN4)-survival”] were considered significant in the UALCAN portal. After adjustment for MOT, this number was reduced to 130 significant, gene variables. In the OCCAMS dataset, 233 gene variables were considered significant following adjustment for MOT.
Expression analysis
Of the 275 t-tests carried out on mRNA-expression levels in tumor versus normal tissue, 136 were statistically significant in UALCAN; after adjustment for MOT, this number was reduced to 118 (42.9% of the genes in the UALCAN dataset). Of the 271 heat-map-related t-tests carried out in OCCAMS, 182 (67.2%) were considered statistically significant following adjustment for MOT. Seventy-five of these genes (75/118 in UALCAN and the same 75/182 in OCCAMS) were differentially expressed across both datasets: 68 were upregulated compared to normal tissue; 4 [homer scaffolding protein 2 (HOMER2), CACNA2D3, voltage-gated Ca2+ channel, L-type, β 4 subunit (CACNB4), and SLC9A4] were downregulated; and 3 [SLC26A9, voltage-gated Ca2+ channel auxiliary subunit gamma 4 (CACNG4), and two-pore segment channel 2 (TPCN2)] were differentially expressed in opposing directions in the two datasets.
The 271 genes presented in the heat-maps have been grouped into the following distinct functional categories: acid-sensing channels, receptors, and their accessory proteins; proton-regulating proteins (Figure 2A and B); Ca2+ pumps and exchangers (Figure 3); Ca2+ channels (Figure 4); Ca2+-release channels and their accessory proteins (Figure 5); transducers (Figure S1); mitochondrial-associated, Ca2+-toolkit genes (Figure S2); cytosolic Ca2+-sensors and -buffers (Figure S3); Ca2+-dependent chaperones (Figure S4); and Ca2+-dependent effectors (Figure S5).
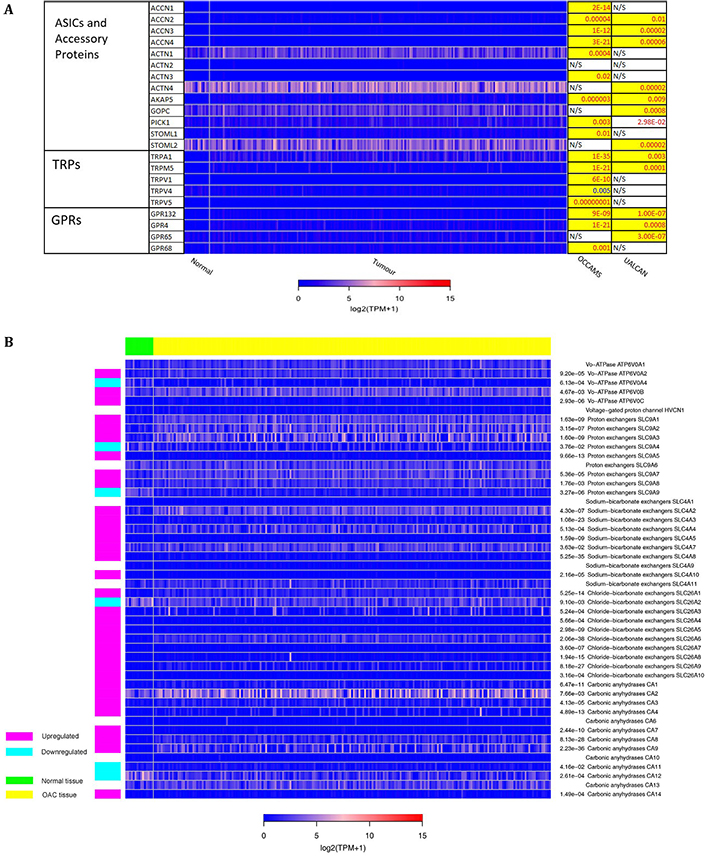
Expression in OAC of A) Acid-sensing channels, receptors and their accessory proteins; and B) proton-regulating proteins. Heat maps depicting the expression levels of genes encoding proteins involved in acid-sensing and proton homeostasis in OAC tumor versus normal tissue. The heat map shows expression data for patients from the OCCAMS dataset. The look-up table represents expression in TPM, with log2 (TPM + 1) scaling. The P-values for significant expression levels (significance was set at P < 0.05) from each dataset are listed on the right, with P-values in red font indicating upregulation, and P-values in blue font indicating downregulation, compared to normal tissue. The P-values highlighted in yellow were considered significant after adjustment for MOT. N/S indicates a non-significant change in expression levels in tumor versus normal tissue. GRPs: ground rubber particles; ACTN: actinin α; AKAP: A-kinase anchoring protein; GOPC: golgi-associated PDZ and coiled-coil motif-containing; PICK: protein interacting with C kinase; STOML: stomatin-like; NHE: Na-H exchange; NHX: Na+/H+ antiporte
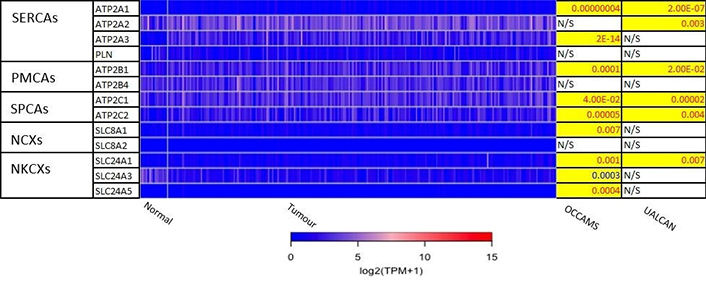
Expression of Ca2+ pumps and exchangers in OAC. Please see Figure 2 for details. NCXs: Na+/Ca2+ exchangers; NKCXs: sodium potassium calcium exchangers; ATP2A: sarco-endoplasmic-reticulum Ca2+ ATPase; PLN: phospholamban; ATP2B: PM Ca2+ ATPases; SLC8A: sodium-Ca2+ exchanger; SLC24A: sodium-potassium-Ca2+ exchanger
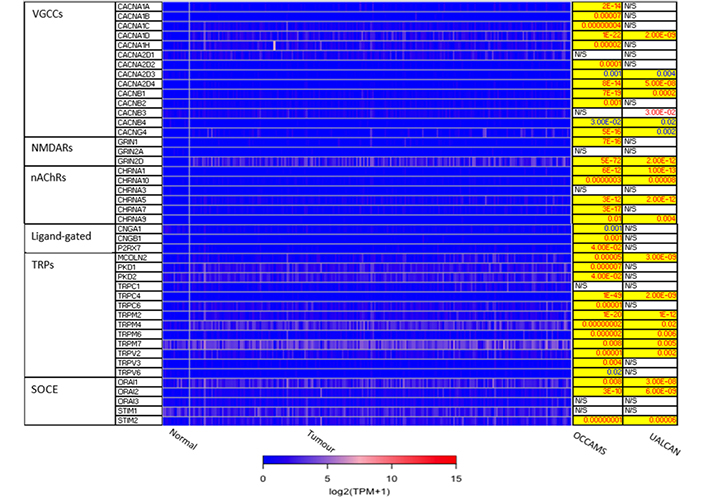
Expression of Ca2+ channels in OAC. MCOLN1 was not available from the OCCAMS data. MCOLN1 was upregulated, compared to normal tissue, in the UALCAN data (P = 9.95E-4). Please see Figure 2 for details. NMDARs: N-methyl-D-aspartate receptors; nAChRs: nicotinic acetylcholine receptors; CNGA: cyclic-nucleotide-gated channel subunit α; CNGB: cyclic-nucleotide-gated channel subunit β; P2RX7: purinergic receptor P2X, ligand-gated ion channel 7; PKD: polycystin; CHRNA: nAChR α
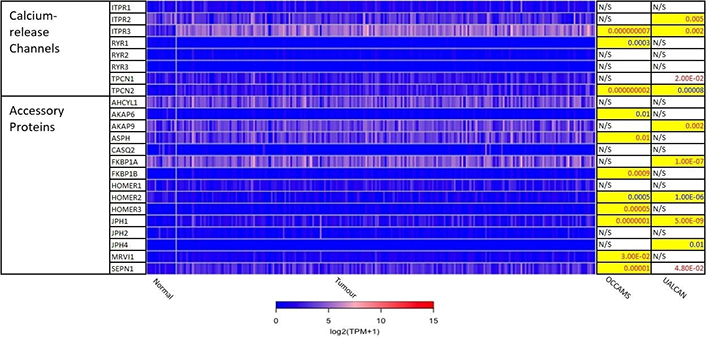
Expression of Ca2+-release channels and their accessory proteins in OAC. JPH3 was not available from the OCCAMS data and was neither significantly upregulated nor downregulated in UALCAN. For details, please see Figure 2. ITPR: IP3 receptor gene; AHCYL: adenosylhomocysteinase-like; ASPH: aspartate β-hydroxylase; CASQ: calsequestrin; FKBP: FK506-binding protein; MRVI1: murine retrovirus-integration site 1 homolog; SEPN1: selenoprotein N1
Further analysis of the 75 genes (significantly differentially expressed across both datasets) revealed that expression of N-methyl-D-aspartate receptor 2D (GRIN2D), TRPC4, and TRPM2 ranked the highest in terms of weighted significance. The individual P-values from the UALCAN data for these genes ranged from between to 2e-9 to 1e-12. The corresponding P-values from the OCCAMS dataset were: GRIN2D, 5e-72; TRPC4, 1e-49; and TRPM2, 1e-20.
Survival analysis and grade and stage expression analysis
Kaplan-Meier log-rank tests on the 271 genes from the OCCAMS dataset revealed that 21 had significant associations with survival (all were associated with improved survival with higher expression). These 21 genes were also analyzed using Kaplan-Meier log-rank tests in the UALCAN portal, but none proved significant. Of the 21 genes significantly associated with survival, 9 had significantly, altered, mRNA-expression levels (as assessed by weighted ranking) in both datasets. The top 6 of these 9 genes were shortlisted for further analysis. Listed in order of their statistical significance (weighted rank of mRNA-expression levels in both datasets), these survival-associated genes were: voltage-gated Ca2+ channel subunit α 1D (CACNA1D), CACNA2D4, junctophilin 1 (JPH1), ACCN4, TRPM5, and secretory pathway Ca2+ ATPase 2 (ATP2C2). Kaplan-Meier survival plots for these 6 genes are shown in Figures 6, 8, 9, 11-13, respectively. CACNA1D, JPH1, and ATP2C2 were also consistently upregulated in various OAC grades and metastatic stages across both datasets. ANOVA boxplots for CACNA1D, JPH1, and ATP2C2 are shown in Figures 7, 10, and 14, respectively. CACNA2D4 was consistently upregulated in various metastatic stages across both datasets, but not in OAC grades (Figure S6). ACCN4 was consistently upregulated in various OAC-tumor grades across both datasets, but not in OAC metastatic stages (Figure S7). TRPM5 only showed significant upregulation in OAC-tumor grades and metastatic stages in the OCCAMS dataset (Figure S8). Despite the importance of the mitochondrion in Ca2+ signalling, we did not find any association of the 13 genes encoding mitochondrial proteins examined with survival outcomes.
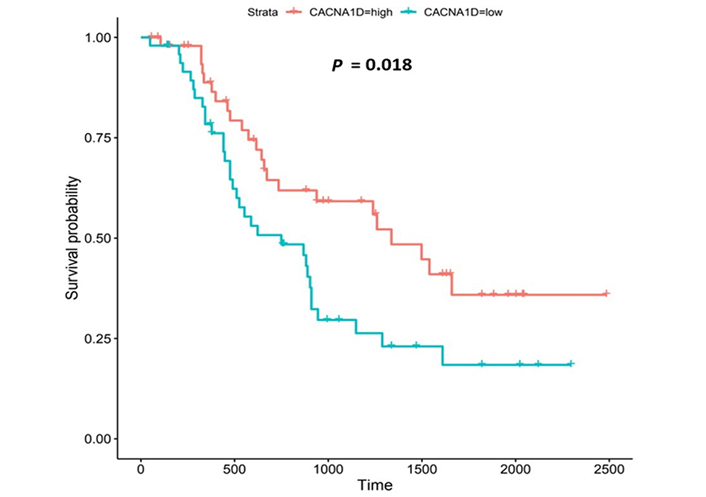
Kaplan-Meier survival plot for CACNA1D. Kaplan-Meier, survival plot (OCCAMS data) for CACNA1D. The comparison was made between patients with high CACNA1D-expression (TPM above the upper quartile) and those with low expression (TPM below the lower quartile). The plot shows survival probability with increasing time in days. A log-rank P-value of < 0.05 was considered statistically significant. An adjustment for MOT (FDR Method [83]) was made
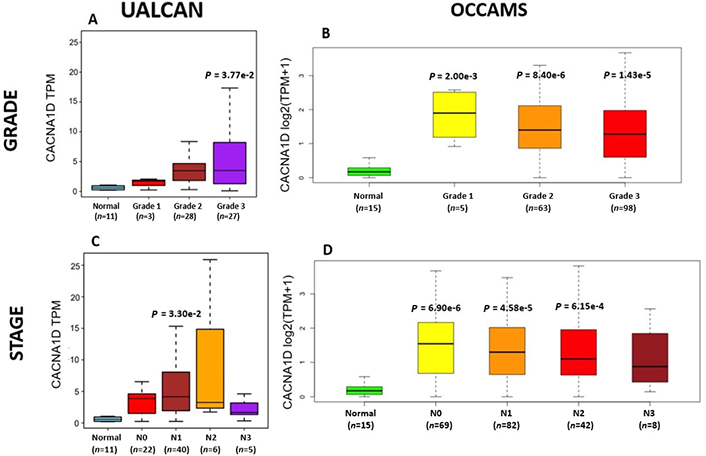
OAC tumor grade and nodal metastatic stage boxplots for CACNA1D. A). (UALCAN data) and B). (OCCAMS data) show CACNA1D-expression across various OAC-tumor grades, compared to normal tissue. C). (UALCAN data) and D). (OCCAMS data) show CACNA1D-expression across various nodal-metastatic stages, compared to normal tissue. A P-value of < 0.05 was considered statistically significant for the boxplots. Tukey HSD post hoc tests were adjusted for MOT. The number of tumor tissue, normal tissue (OCCAMS), or NAT (UALCAN) samples is represented by n
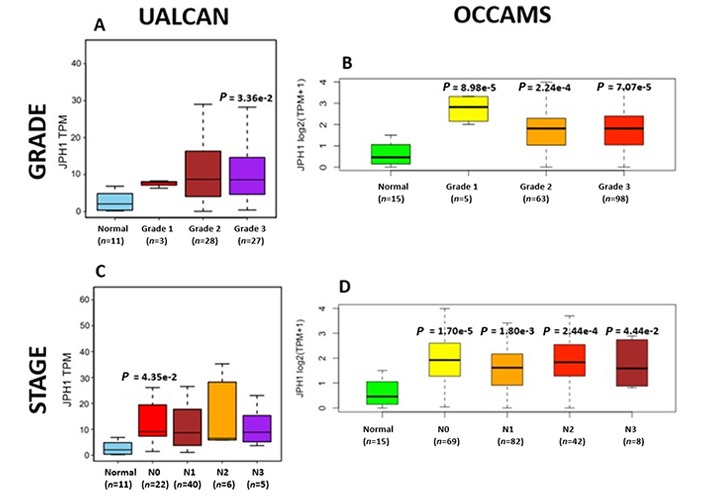
OAC tumor grade and nodal metastatic stage boxplots for JPH1. Details as described in Figure 7
CACNA1D
CACNA1D encodes the α1D subunit of the L-type VGCC or Cav1.3. The channel mediates the influx of Ca2+ into the cell, upon membrane depolarization [85]. It is found in smooth, muscle cells, skeletal muscle, ventricular myocytes, bone (osteoblasts), the brain, the kidneys, the pancreas, the ovaries, the retina, and the cochlea [85, 86]. Patients with higher CACNA1D-expression lived longer than those with lower expression levels (log-rank P = 0.018), Figure 6. CACNA1D-expression was upregulated in Grade 3 versus Normal (P = 3.77e-2) in the UALCAN portal and in Grades 1, 2, and 3 versus Normal in the OCCAMS dataset (P = 2.00e-3, P = 8.40e-6, and P = 1.43e-5, respectively), Figure 7. CACNA1D-expression was upregulated in the N1, nodal-metastatic stage versus Normal (P = 3.30e-2) in the UALCAN portal and in Stages N0, N1, and N2 in the OCCAMS dataset (P = 6.90e-6, P = 4.58e-5 and P = 6.15e-4, respectively), Figure 7.
CACNA2D4
CACNA2D4 encodes the α2 and δ4 subunits of the L-type VGCC, Cav1.4. Like Cav1.3, the Cav1.4 channel mediates the influx of Ca2+ into the cell upon membrane depolarization [87]. Patients with higher CACNA2D4 expression lived longer than those with lower expression (log-rank P = 0.018), Figure 8.
JPH proteins
The JPH proteins contribute to [Ca2+]c homeostasis by forming junctional membrane complexes [88]. Patients with higher JPH1-expression lived longer than those with lower expression (log-rank P = 0.019), Figure 9. JPH1 was upregulated in various OAC-tumor grades in both datasets (Grade 3 versus Normal, P = 3.356e-2 for UALCAN and in Grades 1, 2 and 3 versus Normal, P = 8.98e-5, P = 2.24e-4 and P = 7.07e-5 for OCCAMS), Figure 10. JPH1 was upregulated in various OAC nodal-metastatic stages in both datasets (N0 versus Normal, P = 4.35e-2 for UALCAN and N0, N1, N2 and N3 versus Normal, P = 1.70e-5, P = 1.80e-3, P = 2.44e-4 and P = 4.44e-2 for OCCAMS), Figure 10.
ACCN4
ACCN4 encodes the amiloride-sensitive, cation channel, ASIC4, which has been linked to synaptic transmission, pain perception, and mechano-perception [89, 90]. Patients with higher ACCN4-expression lived longer than those with lower expression (log-rank P = 0.0085), Figure 11.
TRPM5
TRPM5 is a member of the TRP superfamily of ion channels [91]. Patients with higher TRPM5 expression lived longer than those with lower expression (log-rank P = 0.026), Figure 12.
ATP2C2
ATP2C2 encodes an ATPase which transports Ca2+ and Mn2+ into the Golgi lumen to regulate protein sorting, processing, and glycosylation [92]. Patients with higher ATP2C2-expression lived longer than those with lower expression (log-rank P = 0.0018), Figure 13. ATP2C2 was upregulated in various OAC-tumor grades in both datasets examined (Grade 2 versus Normal, P = 2e-2 for UALCAN and in Grades 1, 2 and 3 versus Normal, P = 9.6e-3, P = 3.8e-5 and P = 4.3e-4 for OCCAMS), Figure 14. ATP2C2 was also upregulated in various nodal-metastatic stages in both datasets (N0 versus Normal, P = 5e-2 for UALCAN and in N0, N1 and N2 versus Normal, P = 3e-4, P = 1e-3 and P = 8e-3 for OCCAMS), Figure 14.
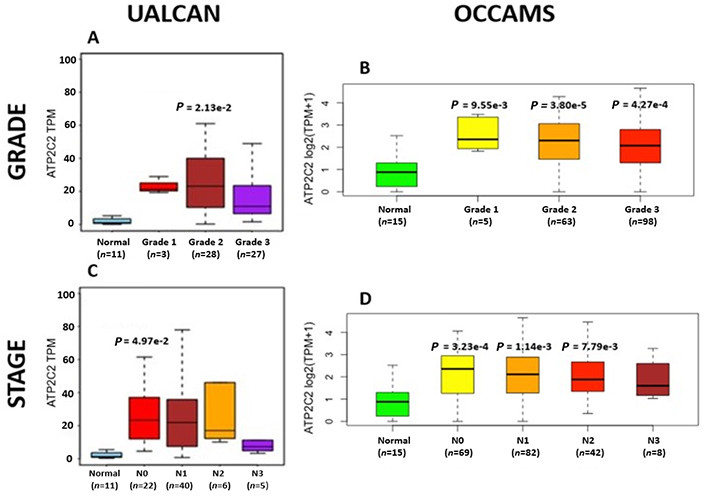
OAC tumor grade and nodal metastatic stage boxplots for ATP2C2. Details as described in Figure 7
Discussion
The goal of the present study was to examine whether there are alterations in the Ca2+ toolkit during the progression of OAC and if altered gene expression is associated with patient outcome. We particularly focused on Ca2+-toolkit proteins involved in acid-sensing and on Ca2+-toolkit proteins located in the mitochondrion. Ca2+ is a key second messenger which regulates most aspects of cell biology, including gene transcription, cellular secretion, cellular motility, and cell death [12]. In cancer cells, dysregulated Ca2+-signalling can lead to transformation, proliferation, and migration [21]. Increases in [Ca2+]c can be elicited by decreases in extracellular pH [10]. During acid reflux, the pH of the distal esophagus can drop from about pH 6.5 to approximately pH 2 [93]. EA acts as a signal for the transformation of squamous to glandular cells in BO, a precursor to OAC [94]. The potential link between Ca2+ signalling and oncogenesis in OAC, and if such oncogenesis can be stimulated by EA, is largely unexplored and offers opportunities for the development of new chemotherapeutic approaches. Such strategies would target EA-related pathways in both pre-malignant disease and OAC.
The present transcriptomic analysis employed two different patient datasets. Our key findings are that CACNA1D, CACNA2D4, JPH1, ACCN4, TRPM5, and ATP2C2 were significantly associated with improved survival and were also significantly upregulated compared to normal tissue in both datasets. Furthermore, there were significant differences in the transcription levels of CACNA1D, JPH1, and ATP2C2 between various tumor grades and nodal-metastatic stages in both datasets. Finally, GRIN2D, TRPC4, and TRPM2 were the most differentially expressed genes, based on weighted rank for significance-level, in both datasets.
CACNA1D encodes the α1D subunit of the L-type VGCC, Cav1.3. Currently, there is no evidence in the literaturelinking CACNA1D toacid-sensing. CACNA1D-expression was upregulated compared to normal tissue in both datasets investigated in the present study. Furthermore, high CACNA1D-expression was associated with improved survival outcomes. CACNA1D-expression was upregulated in Grade 3 versus normal tissue in the UALCAN portal and in Grades 1, 2, and 3 versus normal tissue in the OCCAMS dataset. CACNA1D-expression was upregulated in the second (N1) nodal-metastatic stage compared to normal tissue in the UALCAN portal and in the N0, N1, and N2 stages in the OCCAMS dataset. Other CACNA1D-expression-related studies have shown conflicting results on the effect of levels of this gene in cancers. Most studies have been carried out on prostate cancers, which contain the transmembrane protease, serine 2, gene-erythroblast transformation-specific-related gene (TMPRSS2-ERG) gene fusion. A bioinformatics meta-analysis of the Oncomine dataset (Wang et al. [95]) revealed that various subtypes of VGCCs (CACNA1D or Cav1.3 included) were implicated in the development and progression of diverse types of cancer, including cancer of the prostate, breast, colorectum, bladder, stomach, lung, brain, uterus and esophagus [95]. The same study reported low CACNA1D-expression in sarcoma and renal tumors. Biasiotta et al. [96] noted that CACNA1D showed significantly increased expression in at least 13 of the 25 bladder-cancer datasets analyzed; these bladder-cancer results reinforce those from the meta-analysis by Wang et al. [95]. A Ca2+-toolkit-specific transcriptomic analysis (Pérez-Riesgo et al. [97]) revealed that CACNA1D-expression was increased 1.55-fold in human colorectal cancer cells, compared to normal colon cells. In endometrial carcinoma, CACNA1D was also upregulated [98]. In a study of radical prostatectomy patients, CACNA1D-expression was correlated with a higher Gleason score (a grading system for prostate cancer) and biochemical recurrence [99]. An analysis of the Oncomine dataset revealed that CACNA1D-expression was significantly higher in prostate cancers with the ERG-gene fusion, compared with the cases without this gene fusion [100]. Jhavar et al. [101] observed that CACNA1D was among the top ten differentially-expressed genes in the ERG-subtype of prostate cancer, compared to samples lacking ERG-expression. Setlur et al. [102] identified CACNA1D as part of an 87 gene signature for ERG-fusion-bearing prostate cancer. An epigenomic-profiling study of prostate cancer tumors noted that CACNA1D was among the top-ten-ranked differentially-methylated (hypomethylated) genes in tissues with ERG fusion, compared to those without [103]. CACNA1D, mRNA-expression was also inversely correlated with methylation of the gene [103]. Phan et al. [104] used Oncomine to calculate the changes in mRNA-expression of VGCCs in 20 types of cancer: in contrast to our findings, CACNA1D exhibited low expression in the brain, kidney, and lung tumors [104]. Analyses relating to the Cav1.3 protein are consistent with our gene-expression findings. Fourbon et al. [105] demonstrated that the Cav1.3 protein was more abundant in colorectal-cancer biopsies, compared to normal tissue. Chen et al. [100] noted that the Cav1.3 protein was more abundant in prostate cancer and modulated androgen receptor transactivation. Furthermore, the use of Ca2+-channel blockers (dihydropyridines, phenylalkylamines, and benzothiazepines) was associated with a reduced risk for a higher Gleason score and ERG-positive prostate cancer [106]. Two studies focused on the association of CACNA1D with survival outcomes [107, 108]. Wang et al. [107] identified CACNA1D as part of an 18-ion-channel, prognostic-gene signature in glioma (the direction of the association with survival outcomes was not reported); this finding was observed even though CACNA1D-expression was downregulated in these cells. Xing et al. [108] noted that CACNA1D was one of 8 out of 3,747 differentially-expressed genes associated with survival outcomes in colon adenocarcinoma: consistent with our findings, those with higher CACNA1D-expression lived longer than those with lower expression [108]. Similar to our metastasis-related findings, CACNA1D was among the 6 genes associated with tumor node metastasis staging in colon adenocarcinoma [108]. Additionally, Fourbon et al. [105] showed that colon cancer cell migration was affected by CACNA1D-expression: the migration was decreased when CACNA1D was silenced.
The observation that CACNA1D was upregulated in OAC, but was also associated with improved patient survival, is of interest. Such associations with improved survival may be due to the potential effect of CACNA1D on Ca2+-dependent cancer-cell death. VGCC-associated (particular Cav1-associated), Ca2+-dependent cell death has been well described in pancreatic β cells [109], but to date, not in OAC. Several cancer-predisposing mechanisms have been linked to VGCC function, including the direct influx of Ca2+ into the cell, the involvement of VGCC subunits, and the involvement of the steroid 17β-estradiol [98, 105, 110]. Ca2+ can enter the cytoplasm from the ER through the interaction between VGCCs and the RyR1, a process which facilitated by JPHs [111, 112]. The role of VGCC accessory subunits has been described in the mechanistic paragraph for CACNA2D4 below. The potential role of 17β-estradiol in VGCC-associated carcinogenesis has been studied in endometrial carcinoma. Specifically, upregulation of CACNA1D was associated with increased proliferation and migration in endometrial carcinoma tissue. These effects were enhanced by 17β-estradiol, via the G protein-coupled estrogen receptor [98]. A previous study showed that these estrogen-stimulated effects were decreased by Ca2+-channel blockers (nifedipine and mibefradil) [110]. It is possible that any of the cancer-predisposing mechanisms of VGCCs mentioned could be inactivated in OAC, particularly if such inactivation was acid-dependent. Such inactivation would be consistent with the improved patient survival observed in the present study.
The CACNA2D4 gene encodes the α2 and δ4 subunits of VGCC complexes and has been reported to be an oncogene [113]. CACNA2D4-expression was upregulated compared to normal tissue in both OAC datasets. High CACNA2D4 expression was also associated with improved survival outcomes. CACNA2D4 was upregulated compared to normal tissue in the fourth (N3), nodal-metastatic stage of OAC in the UALCAN portal, and in the first (N0) and third (N2) nodal-metastatic stages in the OCCAMS dataset. There is a paucity of literature investigating the role of CACNA2D4 in OAC and in acid-sensing. A DNA-methylation study showed that CACNA2D4 mRNA expression was upregulated in cultured gastric cancer cell lines, compared to normal stomach cells [87]. The role of CACNA2D4 in pancreatic adenocarcinoma was examined by Xu et al. [114], using data from TCGA: in contrast to our findings, the authors noted a poor prognosis in a subset of patients with high CACNA2D4-expression. An analysis of 98 Ca2+-regulating genes from two gene-expression-profiling datasets on gastric cancer, highlighted that CACNA2D4 was associated with either a 40% decrease (Dataset One) or a 2.9-fold increase (Dataset Two) in overall survival [41]. Similar to our findings, CACNA2D4 was one of the few genes associated with the metastasis of uveal melanoma [115].
Interestingly, the related CACNA2D3 gene (encoding α2 and δ3 subunits) was downregulated in OAC tissue compared to normal tissue, in both the UALCAN and OCCAMS datasets; we did not, however, identify an association between CACNA2D3-expression and survival. Like CACNA2D4, research into the role of CACNA2D3 in OAC and in acid-sensing is lacking. However, there have been a number of studies on OSCC: Li et al. [116] reported tumor-suppressor activity of CACNA2D3 in OSCC cell lines and demonstrated that decreased expression in OSCC patients was associated with poor survival and enhanced metastasis. Increased CACNA2D3-expression has also been linked with enhanced chemosensitivity of OSCC to cisplatin [117]. An association between CACNA2D3 and both patient survival and tumor metastasis has been demonstrated in other cancers [87, 118]. High CACNA2D3-expression was associated with improved survival outcomes in advanced gastric cancer: patients with detectable CACNA2D3 gene methylation had a significantly shorter survival time than patients without this methylation [87]. Methylation-dependent transcriptional silencing of CACNA2D3 was shown to contribute to the metastatic phenotype of estrogen-receptor-positive primary breast cancer, again illustrating a protective nature of the gene [118]. We did not perform a metastasis-related analysis for CACNA2D3 in our study because it did not show any significant association with survival. However, it could be useful to investigate whether CACNA2D3 is a tumor suppressor in OAC. It would also be valuable to know whether there are any synergistic effects between CACNA2D4 and CACNA2D3; the literature points to each gene having opposing roles in the cancers.
The observation that CACNA2D4 upregulation was associated with improved patient survival warrants further study. The increased expression of VGCC accessory subunits, α2δ and β, has been related to different cancer hallmarks in liver, ovarian, prostate, pancreatic, lung, and colon tumors [119]. Supporting our observations, Wang et al. [113] showed that CACNA2D4 played a role in mitigating the adverse effects of first-line chemotherapy (adriamycin or cisplatin) in the treatment of gastric cancers overexpressing bromodomain-containing protein 9 (BRD9). A mechanistic study has been carried out on CACNA2D3. Li et al. [116] demonstrated that CACNA2D3 inhibited tumorigenicity by arresting the cell cycle at the G1/S checkpoint, through increased p21 and p53 expression. Li et al. [116] also demonstrated that CACNA2D3 inhibited cell motility and induced Ca2+-dependent apoptosis. Similar to the CACNA2D4 observations of Wang et al. [113], Nie et al. [117] noted that increased expression of CACNA2D3 enhanced the chemosensitivity of OSCC to cisplatin via Ca2+-mediated apoptosis and the suppression of the phosphoinositide 3-kinase/protein kinase B (PI3K/Akt) pathway. The adverse effect of CACNA2D3-methylation (CACNA2D3-downregulation) has been described [87, 118]. Elucidating the effects of CACNA2D4 on tumorigenicity, cell motility, apoptosis and chemosensitivity would be an invaluable addition to Ca2+ and OAC literature and would consolidate CACNA2D3-related literature.
JPH1 encodes the JPH1 protein [4]. The JPH proteins contribute to [Ca2+]c homeostasis by forming junctional membrane complexes; this is achieved by anchoring the Sarco-/Endoplasmic reticulum to the PM [4]. JPH1 and JPH2 are abundant in skeletal muscle and their suppression leads to the disruption of the activity of SOCE [88]. In our study, high JPH1-expression was associated with improved patient survival. Additionally, JPH1-expression was upregulated compared to normal tissue across both the UALCAN and OCCAMS datasets. Further analysis revealed that JPH1 was upregulated across advanced OAC-tumor grades and OAC nodal-metastatic stages. There is no literature linking a role for JPH1 in OAC or acid-sensing. JPH1 was among the upregulated genes in an analysis of lung cancer [120]. Zou et al. [121] noted that the long non-coding form of JPH1 RNA, Lnc-JPH1-7, was upregulated 35-fold in TCGA samples of head-and-neck, squamous-cell carcinoma. Low expression of this long non-coding RNA promoted survival, consistent with it suppressing the expression of the coding form of JPH1 [121]. A study by Tsantoulis et al. [122] on uveal melanoma demonstrated that JPH1-expression was associated with relapse. By contrast, in uveal-melanoma tissue, JPH1 expression was downregulated compared to normal tissue [123]. An analysis by Que et al. [124] revealed that JPH1 was one of 14 mRNA transcripts involved in regulating a microRNA-circRNA network of genes likely involved in the development or prevention of colorectal cancer [124]; whether JPH1-expression was upregulated or downregulated was not reported in this analysis. A mutation in the JPH1 gene was noted in a patient with human T-lymphotropic virus type-1 (HTLV-1)-associated myelopathy/tropical spastic paraparesis who subsequently developed adult T-cell leukemia [125]. JPH1 was among the top 20 genes associated with survival in endometrial carcinoma [126]; whether JPH1 was associated positively or negatively with survival was not reported. Consistent with our findings, JPH1 was one of 14 of 7,222 genes identified as being strongly associated with a better prognosis in squamous-cell lung carcinoma [127]. Again, Zou et al. [121] noted associations between elevated Lnc-JPH1-7-levels and head-and-neck squamous-cell carcinoma: this time a link with poor prognosis was observed. The authors also highlighted a significant correlation between Lnc-JPH1-7 and the advanced, tumor stage [121]. Metastasis-related literature also aligns with our findings. Tsantoulis et al. [122] demonstrated that the expression of a combination of JPH1 and the protein-tyrosine phosphatase 4A3 (PTP4A3) gene correlated with an increased risk of developing liver metastasis in colorectal and breast cancer (hormone-positive tumors only) [122]. Zou et al. [121] illustrated how short hairpin RNA-mediated knockdown of Lnc-JPH1-7 reduced the expression of epithelial-mesenchymal-transition-promoting genes in head-and-neck squamous-cell carcinoma cell lines.
Similar to CACNA1D and CACNA2D4, high JPH1-expression was associated with improved patient survival in our OAC study. A specific microRNA, miR-145, is the most compelling proposed underlying mechanism for this association with survival. miR-145 has been shown toregulate tumorigenesis, proliferation, differentiation, apoptosis, metastasis, angiogenesis, and therapeutic resistance in certain cancers [128, 129]. It has also been downregulated compared to normal tissue in several cancers, including OSCC. If upregulated, as in the case of OAC, miR-145 is typically accepted as a tumor-suppressor and a suppressor of therapeutic resistance [129]. Xu et al. [128] noted that JPH1 was one of 78, potential targets of miR-145. If in the present study, JPH1 is upregulated in OAC and associated with improved survival, it would suggest that miR-145 is interacting with JPH1 post-transcriptionally to favor tumor suppression. Although calmodulin-dependent protein kinase 1D (CAMK1D) and calmodulin-dependent protein kinase 2D (CAMK2D) were also targeted by miR-145 in the study by Xu et al. [128], these proteins exhibited little significance in the present study. Other studies have focused on JPH2 and JPH3. JPH2 was among 10 individual genes of a DNA-methylation signature associated with overall survival of gastric cancer patients [130]: in contrast to the JPH1 observations of the present study, higher JPH2-methylation (gene-downregulation) was associated with longer survival [130]. In lung adenocarcinoma, JPH3 was downregulated 0.2-fold by S100A2 (associated with favorable prognosis in p53-negative tumors) and 0.43-fold by S100A4 (associated with poor prognosis in p53-positive tumors) [131]. The potential role of the upregulation of S100A2 and S100A4 in the downregulation of JPH1 was not consistently observed in the present study. Again, laboratory experimentation is required to verify the roles of JPH1 in OAC.
ACCN4 encodes ASIC4 [6]. ASIC4 is thought to regulate other members of the ASIC family, particularly in the generation of pain-related currents [89]. ASICs 1a, 1b, 2a and 3 all sense transient and sustained acidification [90, 132]. In our study, we noted that ACCN4 was upregulated in both the UALCAN and the OCCAMS datasets. High ACCN4-expression was associated with improved survival outcomes. Further analysis revealed that ACCN4 was upregulated compared to normal tissue in advanced OAC-tumor grades (both datasets), and in the first (N0) nodal-metastatic stage in the OCCAMS dataset. There is no literature linking ACCN4-expression with OAC. Other cancer-related literature focuses on ACCN4-expression and metastasis, the results of which are conflicting. A study by Marques et al. [133] found that ACCN4 was upregulated by r1881 (a synthetic androgen) in hormone-therapy-resistant prostate cancer cell lines. By contrast, ACCN4 was overexpressed in cisplatin-sensitive ovarian cancer cells [134], suggesting a protective role of the gene. ACCN4-downregulation in head-and-neck squamous-cell carcinoma was noted by Braakhuis et al. [135]. A polysaccharide from the marine algae, Gracilariopsis lemaneiformis, (known for its anticancer activity) significantly decreased ACCN4 transcription in a lung-cancer cell line [136]. In an analysis of the Oncomine dataset, focusing on 5 histologically distinct solid tumors (bladder cancer, glioblastoma, melanoma, breast, invasive-ductal cancer, and lung carcinoma), ACCN4-expression was neither upregulated nor downregulated, compared to normal tissue [96]. There is a paucity of literature associating ACCN4-expression with survival outcomes. Two studies highlighted a role for ACCN4 in metastatic tissue (one indicating a positive association and one indicating a negative association) [135, 137]. Di Pompo et al. [137] investigated whether breast cancer metastasis-induced, bone pain was associated with the effect of EA acting on the mesenchymal, tumor-associated stroma. The authors used human osteoblast primary cultures from healthy donors and cancer-associated fibroblasts from the tumor biopsies of patients with metastasis [137]. After exposure of both types of cells to a medium at pH 6.8 for 6 h, they noted increased mRNA expression of ACCN4 and GPR65 [137]. They concluded that bone metastasis-associated mesenchymal cells have mechanisms in place to perceive the acidification of the metastasis microenvironment, leading to pain and that such findings may have implications for breast cancer palliative care [137]. The authors did not, however, establish whether ASIC4 sensed the EA, either alone or in conjunction with GPR65. In contrast to the study by Di Pompo et al. [137], Braakhuis et al. [135] noted that ACCN4-expression was downregulated in metastasized head-and-neck squamous-cell carcinoma compared to non-metastasized tissue.
ACCN4-upregulation led to improved survival outcomes in OAC in the present study. This is particularly interesting as the gene family has an established link to acid-sensing [90, 132]. Relative to other ASICs, however, literature on ACCN4-function is scarce. Zhou et al. [138] examined the molecular effects of ACCN1, 2, 3, and 4 and noted that only ASIC2 (encoded by ACCN1) promoted invasion and metastasis of colorectal cancer, under acidosis; such metastasis was achieved by the activation of the calcineurin/NFAT1 axis [8]. The subtype of the calcineurin gene protein phosphatase 3 catalytic subunit alpha isoform (PPP3CA) and the NFATC1 gene was upregulated only in the OCCAMS dataset in the present study. Zhang et al. [139] showed that ASIC1 channels promote the growth of gastric cancer by upregulating autophagy. It would be interesting to expand on this study and investigate the potential effects of other ASICs on autophagy in cancer.
TRPM5 encodes a voltage-sensitive monovalent cation-selective channel, which is activated by elevated Ca2+ [91, 124]. EA can also influence TRPM5-activity [140]. Decreases in extracellular pH either quickly block TRPM5-induced current (IC50 at pH 6.2) or slowly enhance current inactivation [140]; the former is reversible while the latter is irreversible [140]. Our findings show that high TRPM5-expression was associated with improved survival outcomes in OAC. TRPM5-expression was upregulated compared to normal tissue in both the UALCAN and OCCAMS datasets. It was also upregulated in various OAC tumor grades and nodal metastatic stages in the OCCAMS dataset only. There are no studies in the literature examining the role of TRPM5 in OAC. In an mRNA-expression analysis of bladder carcinoma patients, Ceylan et al. [141] reported significant reductions in TRPM5-expression in patient tissue. TRPM5 was hypomethylated in OSCC compared to normal tissue [142]; whether such hypomethylation led to increased TRPM5-mRNA expression was not reported. When we examined the UALCAN portal for TRPM5-expression in OSCC tumor tissue, we noted no significant difference, compared to normal tissue. In a pan-cancer analysis by Qin et al. [143], no significant differences in TRPM5 transcripts were observed between normal tissue and breast cancer, lung cancer, and colorectal cancer samples. In a hospital-based case-control study on nucleotide polymorphisms in childhood leukemia, it was observed that patients with the CG or GG genotype of the rs2301696 location in TRPM5 had a decreased risk of developing childhood leukemia, compared to those with the CC genotype [144]. One study examined the association of TRPM5 with patient survival in various cancers. In contrast to our findings in OAC, high TRPM5-mRNA expression correlated with poor overall survival in patients with melanoma and gastric cancer [50]; high TRPM5-expression did not, however, correlate with poor, overall survival in patients with ovarian, lung, breast, or rectal cancer [50]. In agreement with our findings from the OCCAMS dataset, TRPM5-expression has been implicated in metastasis [50, 51]. Sutoo et al. [51] noted that the adaptation of lung cancer cells to chronic acidic extracellular conditions (pH 6.2) elicited a sustained increase in lung cancer cell invasion and metastasis, with TRPM5 being expressed in these cells. Similarly, in murine B16-BL6 melanoma cells, TRPM5 mediated acidic extracellular-pH signalling, whereas TRPM5 inhibition reduced spontaneous metastasis in these cells [50].
In the current study, TRPM5-upregulation was associated with improved survival outcomes. Literature citing a mechanism underlying the association of TRPM5 with cancer is scarce. The literature which does exist focuses on TRPM5-related mechanisms in the tumor microenvironment (TME), or in cancer metastasis [50, 145, 146]. Mucin, secreted by goblet cells, is needed to form a physical barrier to protect epithelial cells from stress-induced damage (including acid-induced damage). Mitrovic et al. [146] concluded that, in a human colonic cancer goblet cell line, TRPM5 mediated (via the NCX1) the entry of Na+ to the cell; this, in turn, resulted in the uptake of Ca2+ and the secretion of mucin 5AC. The upregulation of TRPM5 in OAC tissue in the present study therefore might protect the lining of the esophagus following exposure to EA. Sakaguchi et al. [145] demonstrated a role for TRPM5 in immune cells: they showed that TRPM5 negatively regulated Ca2+-dependent inflammatory responses [production of IL-6 and chemokine C-X-C ligand 10 (CXCL10)] in B lymphocytes. Some authors have already described the role of immune cells in OAC [147–149]. Again, the upregulation of TRPM5 observed in the present study may protect the esophagus from deleterious inflammatory responses. Maeda et al. [50] established a pathway for TRPM5-mediated, lung metastasis in a murine melanoma cell line: following activation by EA, TRPM5 increased [Ca2+]c; this, in turn, activated nuclear factor kB (NF-kB) which subsequently increased the expression of matrix metalloproteinase-9. Matrix metalloproteinase-9 potentially supports the lung metastasis in this cell line, by degrading collagen in the extracellular matrix [50]. Further study in human tissue is needed to verify these findings.
ATP2C2 encodes an ATPase pump involved in the transport of Ca2+ and Mn2+ into the Golgi [92]. It is also involved in Ca2+ signalling independent of its ATPase activity [92]. In particular, SPCA2 (the protein encoded by the ATP2C2 gene) interacts with the SOCE channel, Orai1, and induces Ca2+ influx at the cell surface [150]. It has been shown that unbalanced, store-independent Ca2+ signalling can lead to enhanced cell proliferation and tumorigenesis [150]. Our findings show that ATP2C2-expression was upregulated in OAC in both datasets. In addition, higher ATP2C2-expression was associated with improved patient survival. Further analysis revealed that ATP2C2 was significantly upregulated in various OAC tumor grades and nodal-metastatic stages in both datasets. ORAI1 (encoding the Orai1 channel) is upregulated in OAC tissue compared to normal tissue in both the UALCAN and OCCAMS datasets, supporting this potential store-independent interaction [150]. Our ATP2C2-expression analysis results are consistent with those of Hyland et al. [151], who analyzed gene expression in BO. Comparison of BO-samples to same-patient, normal mucosa from squamous esophagus revealed a 2.33-fold increase in ATP2C2 expression in BO [151]. Similar observations have been made in breast cancer analyses. In one breast cancer study, ATP2C2 was upregulated compared to normal tissue [152]. In a separate set of breast cancer samples, SPCA2 knockdown enhanced sensitivity to DNA-damaging agents, including doxorubicin, cisplatin, and ionizing radiation [153]. Survival-related observations in the literature contradict our findings. A gene-expression study [152] noted that high ATP2C2-expression was associated with worsened patient survival in OSCC, breast cancer, thyroid carcinoma, head-and-neck squamous cell carcinoma, kidney, renal clear cell carcinoma, and lung squamous cell carcinoma [152]. Liu et al. [152] showed that ATP2C2-expression negatively correlated with patient survival in breast cancer. In a study by Makena et al. [153] on the SPCA2 protein, high abundance was associated with poor prognosis in luminal ER+/PR+ breast cancer subtypes. Similarly, Zhao et al. [154] (TCGA data) revealed a 76% decreased survival rate among thyroid cancer patients with the ATP2C2 gene, compared to those without. Again, in contrast to our OAC findings, Zhang et al. [155] noted that the long-non-coding-RNA version of ATP2C2 (ATP2C2-antisense 1) was associated with worsened overall survival in thyroid carcinoma. Metastasis-related studies show inconsistent results. Similar to our findings, a breast cancer analysis by Liu et al. [152] showed that ATP2C2-expression was correlated with advanced breast cancer stages (the “T” and “N” components). A separate, breast cancer study, however, showed that high SPCA2 levels protected against the initiation of the epithelial-mesenchymal transition [156].
ATP2C2 upregulation was associated with improved patient survival in the present study. There is a lack of data in the literature highlighting improved patient survival with ATP2C2-upregulation, for any cancer. The literature on carcinogenesis points to either an Orai1-related mechanism, extracellular signal-regulated kinase 1/2 (ERK1/2)-activation, the adaptation to hypoxia, or to the influence of ATP2C2 on the TME [92, 152, 157–159]. In OSCC, tumors displayed an increased abundance of the Orai1 protein, and this increased abundance was associated with poor overall and recurrence-free survival; furthermore, pharmacological antagonists of Orai1 reduced OSCC proliferation, invasion, and tumorigenesis [157]. Kohn et al. [158] noted that high SPCA2-abundance was correlated with epithelial genes in cancer cell lines. Similarly, Feng et al. [92] demonstrated that SPCA2-overexpression conferred increased proliferation in a nonmalignant mammary, epithelial cell line. The authors demonstrated that this increased proliferation was due to the activation of the ERK1/2 pathway [92]. Jenkins et al. [159] showed that ATP2C2 helped colon cancer cells adapt to hypoxia, prevented cell death, increased proliferation capacity, and promoted tumor growth. Liu et al. [152] demonstrated that ATP2C2 might be a potential indicator of TME status. Specifically, genes from the patient group with low ATP2C2-expression were significantly enriched in immune-related activities. Genes from the high, ATP2C2-expression group were mainly enriched in metabolic pathways [152]. The low, ATP2C2-expression group had increased numbers of pro-tumor M2 macrophages and decreased numbers of anti-tumor M1 macrophages [152]. The immune cell profile of the TME (of breast cancer in the study by Liu et al. [152]) may therefore override the tumorigenic effects inside the cell and warrants further study. Similarly, the acidic TME of OAC warrants further study and may explain improved survival in OAC patients and not in other cancers.
GRIN2D encodes the 2D subunit of the NMDAR. NMDARs are ligand-gated, glutamate receptors involved in Ca2+ signalling, primarily in neurons [160]. Our data shows that GRIN2D-expression was upregulated in OAC: the gene is the highest weighted-ranking gene in the two datasets examined. Zhang et al. [161] showed that GRIN2D-expression was upregulated in 5 OSCC samples from Chinese patients. Conversely, GRIN2D was among the 13 genes that were hypermethylated (downregulated) in both OAC and OSCC [162]. In meibomian cell carcinoma (cancer of the glands of the eyelids), GRIN2D-expression was also downregulated, compared to normal tissue [163]. In a study of various cancer cell types by Luksch et al. [164], knockdown of GRIN2D did not influence phenotype. There are no reported studies demonstrating a role for GRIN2D in patient survival. The NMDAR-2D subunit has been shown to play a role in breast-to-brain metastasis [165]. Endothelial GRIN2D has been shown to promote angiogenesis in colorectal cancer [166].
TRPC4 encodes a voltage- and ligand-gated cation channel, which alters enzymatic activity and initiates endocytosis and exocytosis [167]. TRPC4 channel activity is potentiated by decreases in pH [168]. Our data shows that TRPC4-expression was upregulated in OAC relative to normal tissue: this gene has the second-highest weighted ranking for expression (TPM) in our study. There is currently no reported evidence linking TRPC4 with OAC. Zhang et al. [169] found in a study of 2,433 cases and 2,433 controls, that the TRPC4 polymorphisms rs9547991 and rs978156 were candidate susceptibility markers for lung cancer in a Chinese population. Subjects carrying at least one variant allele had a 1.29-fold increased risk of developing lung cancer, compared with those carrying no variant alleles [169]. By contrast, TRPC4 has been shown to inhibit the proliferation of renal cell carcinoma cells, after the cells were exposed to englerin A (an anti-cancer substance, found in the bark of the Phyllanthus engleri tree) [170]. Consistent with our findings, there are no data in the literature to suggest a role for TRPC4 in patient survival. TRPC4-activation by GPR68 agonists promoted the invasion and metastasis of granule precursor-derived human medulloblastoma [171]. TRPC4-downregulation has been proposed as a trigger for tumor angiogenesis in renal cell carcinoma [172].
TRPM2 encodes a Ca2+-permeable non-selective cation channel which is activated by adenosine diphosphate ribose (ADP-ribose), increased temperature, oxidative stress, and Ca2+ [173–175]. TRPM2 gating is inhibited at pH 5.5 to 6.7, indicating a role in acid-sensing [176]. Our data shows that TRPM2 expression was upregulated in OAC: the gene has the third-highest weighted rank. There is no evidence linking a role for TRPM2 in OAC; evidence for a role of TRPM2 in other cancers is more extensive. A study of TRP-family-mRNA expression by Qin et al. [143], revealed that TRPM2-expression was upregulated in breast cancer (ductal carcinoma and invasive breast cancer), small-cell, lung carcinoma, colorectal cancer (colon and caecum adenocarcinoma), gastric cancer, and melanoma. Conversely, TRPM2-expression was downregulated in prostate cancer and in the brain and central nervous system cancers [143]. Sumoza-Toledo et al. [177] noted somewhat similar results in breast cancer: TRPM2 was upregulated in invasive breast carcinoma, compared to normal tissue. TRPM2-expression was upregulated in oral squamous cell carcinoma (OrSCC) tissue [178]. Findings from an in vitro study [179] on prostate cancer contradict those from Qin et al. [143]: here, TRPM2 played a key role in prostate cancer proliferation, as demonstrated by small interfering RNA techniques [179]. In non-small-cell, lung cancer, the long non-coding RNA [TRPM2-antisense RNA (AS)] was upregulated, and subsequent downregulation of TRPM2 promoted apoptosis in vitro [180]. In OrSCC, low TRPM2-expression was associated with poorly- or moderately-differentiated, tumor tissue grades [181]. TRPM2-expression also predicted survival outcomes in breast, lung, or colorectal cancers [143]. In contrast to Qin et al. [143], a study by Sumoza-Toledo et al. [177] demonstrated that high expression was associated with improved survival outcomes in both the human epidermal growth factor receptor 2 (HER2)+ and ER, breast cancer subtypes. In contrast to findings reported by Chen et al. [182], Gil-Kluick et al. [183] reported that high TRPM2 expression was associated with improved patient survival in acute myeloid leukemia (AML). Several mechanisms underlying TRPM2 function have been cited. Chen et al. [182] showed that TRPM2 promoted AML proliferation and cellular survival through the modulation of mitochondrial function, ROS, and autophagy. In both in vitro and murine model studies, Almasi et al. [184] suggested that TRPM2 promoted gastric-cancer migration, invasion, and tumor growth through the AKT pathway; the same group previously demonstrated that TRPM2 promoted gastric-cancer cell survival via the c-Jun NH2-terminal kinase (JNK) pathway [185]. Two studies examined the role of TRPM2 in pancreatic ductal carcinoma: the first observed that TRPM2-overexpression promoted cell proliferation, invasion, and migration [186]; the second implicated the protein kinase C/mitogen activated protein kinase pathway as the mechanism by which TRPM2 exerts these effects [187]. TRPM2 was also shown to be essential for the survival and migration of OrSCC [178].
The present study has several limitations. Our analysis is based exclusively on transcriptomic data: this does not account for potential changes in protein function, resulting from altered translational or post-translational mechanisms. Evidence from an ovarian-cancer xenograft model, however, has indicated that the correlation between mRNA and protein abundance is closer for differentially expressed genes than for those whose expression is not altered [188]. A comparative analysis, using a proteomic dataset, would make the results of the present study more robust. The use of NAT, as a source of non-cancerous cells in UALCAN, is also a potential source of error: these cells might potentially be in a cancerous or pre-cancerous state [189]. Indeed, Aran et al. [189] noted that NAT may have properties distinguishing it from both tumor tissue and a more stringent classification of normal tissue; none of the 18 genes highlighted in the study were investigated in the present study. The statistical power of each dataset has an influence on the results obtained. The UALCAN portal compares data from 89 OAC-tumor samples with 11 NAT samples, while the OCCAMS dataset compares data from 213 samples with 15 normal-tissue samples. We note that none of the 21 survival-associated genes from the OCCAMS dataset were significant in UALCAN, after adjustment. We also note that a higher proportion (67.2%) of OCCAMS, heatmap-related t-tests proved significant after adjustment for MOT, than in UALCAN (42.9%). It is possible that the UALCAN portal did not have sufficient power to detect all statistically significant differences.
In conclusion, the present study has implicated 6 genes (CACNA1D, CACNA2D4, JPH1, ACCN4, TRPM5, and ATP2C2) as potential prognostic markers and 3 genes (GRIN2D, TRPC4, and TRPM2) as candidate diagnostic markers for OAC. Of these, ACCN4, TRPM5, TRPC4, and TRPM2 have established roles in acid-sensing, with ACCN4 having an indirect role [140, 168, 176, 190]. With the exception of CACNA1D (OAC-expression data), ATP2C2 (BO-expression data), and GRIN2D (OAC-methylation data), published, OAC-related data is lacking for the genes highlighted by the current study. Higher expression of all 6 prognostic genes in this study was associated with improved survival outcomes. These positive survival observations were noted in conjunction with high expression of all of these 6 genes in OAC tissue, compared to normal tissue, and sometimes (as in the case of CACNA1D, JPH1, and ATP2C2) in advanced metastatic stages and tumor grades. We hypothesize that these genes may be either increasing tumor cell death and/or inhibiting other cancer hallmarks, either directly or by indirect mechanisms. In the literature, the association of CACNA1D with improved survival outcomes in colon adenocarcinoma, and of JPH1 with improved survival outcomes in small-cell lung carcinoma, was particularly strong [108, 127]. The associations of the other genes with survival outcomes in the literature were either inconsistent with those of our study (CACNA2D4, TRPM5, and ATP2C2) or unreported (ACCN4). With the exception of JPH1 (targeted by miR-145 to favor tumor-suppression), CACNA2D4 (mitigation of the adverse effects of chemotherapy in BRD9, gastric cancer), and TRPM5 (the Ca2+-dependent regulation of inflammatory responses and the production of mucin), there are no clear molecular mechanisms cited to explain the improved patient survival observations in the present study. Indeed, most mechanisms cited in other cancers would intuitively lead to worsened patient survival. Because an acidic environment is what differentiates OAC from many cancers, the potential role of EA in OAC is again brought into focus. Three studies have implicated TRPM2 in metastasis. Other studies also consistently linked high expression of CACNA1D, CACNA2D4, JPH1, TRPM5, ATP2C2, GRIN2D, and TRPC4 with metastasis. Further research is required to discern which of these 9 genes could prove useful in OAC prognosis or diagnosis. Given the ubiquitous expression of GRIN2D in OAC and OSCC, TRPC4 and TRPM2 may be more selective, diagnostic markers for OAC. The potential interaction between the following partners in OAC may yield promising results: CACNA1D with Cav subunits, the RyR1 and the G protein-coupled estrogen receptor; JPH1 and miR-145; ACCN4 and GPR65; TRPM5 and NCXs; ATP2C2 and ORAI1; and TRPC4 and GPR68. The role of the TME (particularly acidic and immune components) should also be considered in the design of such experiments. Taken together, the present study lays the groundwork for further exploration of potentially oncogenic and protective Ca2+-signalling pathways in OAC.
Abbreviations
ACCN4: | acid-sensing ion channel 4 |
ACTN: | actinin α |
ANOVA: | analysis of variance |
ASICs: | acid-sensing ion channels |
ATP2A: | sarco-endoplasmic-reticulum Ca2+ ATPase |
ATP2B: | plasma membrane calcium ATPases |
ATP2C2: | secretory pathway Ca2+ ATPase 2 |
BO: | Barrett’s esophagus |
Ca2+: | calcium |
CACNA1D: | voltage-gated Ca2+ channel subunit α 1D |
CACNA2D4: | voltage-gated Ca2+ channel auxiliary subunit α2 δ4 |
CACNB4: | voltage-gated Ca2+ channel, L-type, β 4 subunit |
CAs: | carbonic anhydrases |
CHRNA: | nicotinic acetylcholine receptors α |
EA: | extracellular acid |
ER: | endoplasmic reticulum |
FKBP: | FK506-binding protein |
GORD: | gastroesophageal reflux disease |
GPR: | G-protein coupled receptors |
GRIN2D: | N-methyl-D-aspartate receptor 2D |
GRPs: | ground rubber particles |
IL-1: | interleukin-1 |
IP3: | inositol 1,4,5-trisphophate |
IP3Rs: | inositol 1,4,5-trisphophate receptors |
ITPR: | inositol 1,4,5-trisphophate receptor gene |
JPH1: | junctophilin 1 |
MCOLN1: | mucolipin 1 |
MOT: | multiplicity of testing |
NAT: | normal-adjacent tissue |
NCXs: | Na+/Ca2+ exchangers |
NFAT: | nuclear factor of activated T cells |
NMDARs: | N-methyl-D-aspartate receptors |
OAC: | oesophageal adenocarcinoma |
OCCAMS: | Oesophageal Cancer Clinical and Molecular Stratification |
OrSCC: | oral squamous cell carcinoma |
OSCC: | oesophageal squamous cell carcinoma |
PKD: | polycystin |
PM: | plasma membrane |
ROS: | reactive oxygen species |
RyRs: | ryanodine receptors |
SERCAs: | sarcoplasmic reticulum/endoplasmic reticulum Ca2+-ATPases |
SLC24A: | sodium-potassium-Ca2+ exchanger |
SLC26: | solute-carrier family 26 member |
SLC4A: | solute-carrier family 4 member A |
SLC8A: | sodium-Ca2+ exchanger |
SLC9A: | Solute Carrier 9A |
SOCE: | store-operated Ca2+-entry |
SPCA: | secretory pathway calcium ATPase |
STIM: | stromal interaction molecule |
STOML: | stomatin-like |
TCGA: | The Cancer Genome Atlas |
TGF-β: | transforming growth factor-beta |
TME: | tumor microenvironment |
TPM: | transcripts per million |
TRP: | transient receptor potential |
TRPC4: | transient receptor potential ion channel classical or canonical 4 |
TRPM2: | transient receptor potential ion channel subfamily M member 2 |
TRPV6: | transient receptor potential vanilloid 6 |
VGCCs: | voltage-gated Ca2+ channels |
Supplementary materials
The supplementary material for this article is available at: https://www.explorationpub.com/uploads/Article/file/100263_sup_1.pdf.
Declarations
Acknowledgements
The financial support of Breakthrough Cancer Research, the Higher Education Authority of Ireland, and University College Cork is gratefully acknowledged. Statistical advice from Ms. Kathleen O’Sullivan from the Department of Mathematics at University College Cork is also greatly appreciated.
Author contributions
JJM provided the original conception and design of the study. ALC, JJM, AN, GD, and DSC contributed substantially to the extraction, analysis, interpretation, and presentation of the data. RF supervised the analysis for the OCCAMS dataset. AN and GD worked on data from OCCAMS and DSC analyzed data from UALCAN. TRO’D and SLM provided critical insights on cancer biology. ALC wrote the first draft of the manuscript; JJM, TRO’D, and SLM contributed to sections of the manuscript. AN provided key statistical input. ALC, JJM, AN, GD, and DSC contributed to tables and figures. All authors participated in the revision and read and approved the submitted version.
Conflicts of interest
The authors declare no competing financial interests or other conflicts of interest.
Ethical approval
Not applicable.
Consent to participate
Not applicable.
Consent to publication
Not applicable.
Availability of data and materials
All data used in this manuscript were obtained from either The Cancer Genome Atlas (https://www.cancer.gov/about-nci/organization/ccg/research/structural-genomics/tcga), accessed via the UALCAN portal (http://ualcan.path.uab.edu/cgi-bin/ualcan-res.pl/), or the Oesophageal Cancer Clinical and Molecular Stratification (OCCAMS) dataset (https://www.mrc-cu.cam.ac.uk/research/rebecca-fitzgerald/clinical-studies/occams).
Funding
This work was supported by funding from Breakthrough Cancer Research [Grant No. R19491] to JJM, the Higher Education Authority of Ireland, and University College Cork. These sponsors had no direct part in the design, management, execution, or dissemination of this study.
Copyright
© The Author(s) 2021.