Abstract
Acquired or hereditary epilepsy affects millions of people. Today, the disease is pharmacoresistant in about 30 percent of cases, meaning that the seizures do not come under acceptable control in response to medication. Therefore, there is a great need for the development of novel methods for epilepsy research and treatment. Although in vivo animal models best mimic the clinical features of epilepsy, in vitro models have clear advantages in elucidating the fine details and cellular mechanisms of neurological disorders. In contrast to short-lived experiments in acute brain slices, cell cultures are often chosen as chronic models for antiseizure medication screening and epilepsy research under reduced, well-controlled in vitro conditions that still include all major cell types susceptible to epileptic seizures. Organotypic brain slices or dissociated cells produce spontaneous synchronized epileptiform discharges classified as interictal and ictal-like. In addition, pharmacologically or electrically induced seizures and status epilepticus can be obtained for electrophysiological and imaging experiments. Relatively simple cell cultures of primary rodent neurons provide entry-level models for the initial screening of antiseizure medications and basic epilepsy research. However, more sophisticated human cultures of stem cell-derived neurons offer the possibility of medical studies using the human genotype without the need to obtain brain tissue from patients. As an evolution of this method, programmed differentiation of brain cells is now being used in stem cell therapy for neurological disorders. Overall, cell culture greatly expands the repertoire of methods available to study epileptic disorders and potential cures.
Keywords
Epilepsy, cell culture, neuron, excitability, seizures, electrophysiologyIntroduction
Usually, epileptic disorders are characterized by recurrent seizures of varying intensity and frequency [1], which may be provoked or unprovoked [2]. Strokes, trauma, and hypoxia under genetic predisposition and unfavorable conditions induce significant shifts in the excitability of neurons and neuronal networks, resulting in pathological seizure activity. Seizures develop locally as a focal form of epilepsy, which can progress to a generalized state in which many parts of the brain are involved on a wide scale [3]. Highly synchronized and high-frequency neuronal activity underlies epileptic discharges, which are caused by an increased neuronal input-output relationship and increased excitability [1]. Focal epilepsy is more common and its severity depends on how limited the area of seizure activity is. An important prognostic criterion is the localization of the seizure focus whether it is limited and discrete and how it affects vital areas of the brain [4].
The brain cortex consists of two main types of neurons: excitatory glutamatergic and inhibitory GABAergic [5]. During seizures, neuronal activity gets out of control, resulting in an explosive disruption of the balance of excitation and inhibition in the nervous system [4]. Antiseizure medications generally either downregulate excitation mechanisms or enhance inhibition in the central nervous system.
Culturing neurons in vitro allows obtaining mixed cultures of glutamatergic and GABAergic neurons with electrical activity similar to that of brain neurons in vivo. Reduced in vitro preparations combine the ease of access to drugs and other neuroactive treatments along with the ease of recording the data [6]. In the case of generalized seizures, epileptic activity invades many neurons of the brain and forces them to produce excessive meaningless firing. On the other hand, during treatment, antiseizure medications tend to act non-specifically on neurons by targeting their channels or receptors without taking into account the fine structural organization of brain regions. Therefore, it seems feasible to use a reduced neural tissue system to study the effects of therapy on seizure-affected neuronal networks.
Apart from pharmacological studies, gene therapy is currently being considered as a potential alternative to the surgical treatment of pharmacoresistant epilepsy through the genetic expression of additional channels or proteins aimed at reducing the excitability of the main glutamatergic neurons [7–9]. Because routine methods of gene transfer are widely available and highly efficient in cultured cells, in vitro models of epilepsy appear to be very convenient for initial screening of gene therapy methods in a well-controlled environment to determine the potential therapeutic properties of novel vectors.
In vitro models in the hierarchy of preclinical trials
Consistent with the types and causes of human epilepsy, animal models of epilepsy using pharmacological, electrical, genetic, and other methods have helped investigate mechanisms of epileptogenesis to facilitate the development and screening of new treatments [10]. In vivo models most closely mimic the clinical features of human epilepsy and involve the use of animals in chronic experiments, thus providing the opportunity to reveal behavioral correlates and to test therapeutic effects on seizure prevention [11]. On the other hand, when developing new approaches to epilepsy treatment, multistep testing appears to be the most efficient, with putative drugs first tested in reduced in vitro models and then in more expensive and time-consuming in vivo models [12].
The simplest in vitro models of epilepsy, using acute experiments on rodent (mouse or rat) brain slices, are short-lived (up to a few hours). During slicing, neuronal tissue suffers massive damage that does not heal during the experiment. As a result, these preparations are typically only useful for studying acutely provoked seizures. To overcome these limitations, methods of culturing extracted slices (organotypic cultures) for in vitro testing or culturing dissociated neurons to create an “epilepsy in a petri dish” model have been proposed (Table 1). The organotypic slice culture model preserves much of the original brain architecture, allowing recording of electrical activity (including epileptiform discharges) and efficient drug delivery [13, 14]. Organotypic cultures undergo a rapid process of epileptogenesis and respond to anticonvulsant drugs, including those that suppress seizure discharges but not interictal discharges, with subsequent development of drug resistance [11].
Comparison of rodent and human neuronal models of epilepsy in vitro
Model | Cell source | Advantages | Disadvantages | References |
---|---|---|---|---|
Rodents | ||||
Primary neuronal cultures | Newborn or embryonic brain at late stages | • Affordability and ease of obtaining in a short time (weeks) • Preservation of functional neuronal phenotypes • Mouse genetic lines | • Rodent genotype • Rodent neuronal phenotype • Disordered connections between neurons | [15, 16, 30, 40] |
Organotipic brain slices | Extracted brain of young animals | • Preservation of overall tissue architecture • Preservation of functional phenotypes of neurons • Mouse genetic lines | • Rodent genotype • Rodent neuronal phenotype • Post-traumatic reorganization of axons and synapses | [10, 11, 48, 72] |
Human | ||||
hiPSC-derived neuronal cultures | Stem cells from sick and healthy patients, often fibroblasts | • Human genotype • Donors with inherited disease phenotype and specific mutations | • Special requirements and limitations • Difficulty in differentiating functional neurons and neural networks • Long culturing time scale • Need to co-seed different neuronal subtypes and glia • Disordered, poor, or absent connections between neurons | [17, 18, 57, 61] |
Organotipic brain slices | Brain surgery to excise the focus of epilepsy patients | • Human genotype • Donors with inherited disease phenotype and specific mutations • Preservation of overall tissue architecture • Preservation of functional phenotypes of neurons | • Special requirements and limitations • Fast degradation of neurons • Aged brain tissue • Reorganization of axons and synapses • History of treatment with drugs | [49–51, 73] |
An even greater simplification is the use of a dissociated mouse or rat neuronal culture, in which the arrangement of neuronal networks similar to brain architecture is not preserved, but all major cortical and hippocampal neuronal types are present, including inhibitory GABAergic and excitatory glutamatergic neurons, as well as glia [15]. Similar to organotypic cultures, dissociated cultures develop epileptiform activity and, under certain conditions, status epilepticus [16]. In addition, it is possible to culture not only primary rodent neurons, but also secondary neurons differentiated from human stem cells [17, 18]. It allows testing on cultures of secondary neurons from patients with a specific disease phenotype or with identified genetic mutations that underlie epileptic disorders [7, 19]. Thus, in vitro neuronal cultures greatly expand the possibilities of studying epileptogenesis and its treatment by providing valuable and accessible models for primary screening of antiseizure medications at the cellular and neuronal network level.
Functional assays in cell culture models of epilepsy
In the first stage, antiseizure medications are studied in culture without seizure-like events by recording induced currents or voltage steps and testing the effect of putative drugs on them [20–22]. The standard methods of patch clamp/voltage clamp and drug application are used, which are reviewed elsewhere [23, 24]. These experiments allow us to reveal a broad pharmacological profile of a given drug and how it modulates single-cellular physiology, excitability, and transmembrane currents carried by specific channels.
More epilepsy-relevant assays, however, are experiments that induce/facilitate seizure-like activity at the network level. In this way, the effect of putative antiseizure medications on seizure-like network events can be tested. To induce massive ictal activity and status epilepticus in cultured neurons, modulators or activators of the glutamatergic transmission [6, 11, 25] or inhibitors of the GABAergic transmission or of the K+ channels [26, 27] are used. Often, low-Mg2+ artificial cerebrospinal fluid is used to remove the Mg2+ block of N-methyl-D-aspartate (NMDA) receptors to boost the spontaneous activity of the oscillating network [16, 26, 28–30]. Single-unit activity is usually recorded by whole-cell patch clamp [16, 26], and multi-unit activity is recorded by functional Ca2+ imaging [25, 30] or extracellular potential recording [11, 25, 31]. After induction of ictal activity/status epilepticus, putative antiseizure medications can be tested for their ability to reduce or abolish it [26, 31].
Rodent primary neuronal cultures
Unlike stem cells, mature neurons do not divide, so methods for culturing neurons must first solve this problem. One way to address this issue could be secondary differentiation from other cell types that have stem cell characteristics, such as the well-known noradrenergic cell line PC12, which is derived from a rare rat pheochromocytoma [32]. However, neuron-like cells derived from immortalized cancer cultures exhibit genomic instability [33], which compromises the functional properties of the cells and makes them different from normal neurons, thus hindering the reproducibility of experiments [34, 35].
Primary neuronal cultures differentiated from progenitor cells derived from embryonic or postnatal mice or rats with a relatively short period of embryogenesis (17–21 days) have been used to overcome these limitations. Mouse and rat neuronal progenitor cells lose pluripotency after embryonic days 13.5–14 and are only able to differentiate into neurons, whereas glial progenitors are able to differentiate into oligodendrocytes and astrocytes [36]. Thus, the collection of cellular material up to the age of postnatal days 1 and 2 allows culturing of dissociated primary neurons for up to 4 weeks in vitro, which is the age of sexually mature young rodents. At the same time, the coding ability of neurons and the dynamic characteristics of their action potentials improve by postnatal day 15 but remain lower than those of cortical neurons in animals of the same age in vivo [37].
Spontaneous synchronous oscillations caused by bursts of action potentials are also observed in cultured cortical neurons, despite the lack of sensory inputs in vitro. The frequency of oscillations increases with the development of synapses and correlates with their number [38]. The frequency of the oscillations that reverberate back to the active neurons through the synaptic connections is dependent on the size of the pool of neurons that are engaged in an oscillatory cycle [39]. Apparently, neuronal cultures show relatively low-frequency oscillations due to the limited number of neurons involved, while these oscillations have an epileptiform shape.
To model seizure conditions in vitro, a Mg2+-free solution was used to induce status epilepticus in primary neuronal cultures (Figure 1) [30]. While episodic epileptiform convulsive activity occurring during the lifetime of neurons in vitro does not cause increased cell death, Mg2+ deprivation induces significant mortality of neurons [16]. The induced epilepsy recorded on dissociated culture has many similarities with electroencephalography parameters in epileptic patients, including a strict sequence of events of seizure onset, progression, and termination, accompanied by abnormalities in DNA methylation, increased ratio of glutamatergic to GABAergic synapses, and other functional changes in the neuronal networks [40]. Retigabine, an approved antiepileptic drug, was tested in this model and demonstrated suppression of seizure-like activity induced by low Mg2+ by significantly reducing spontaneous spiking in culture [26].
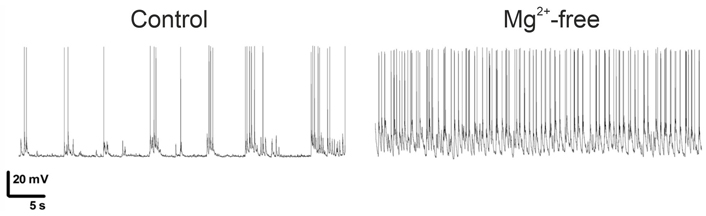
Low levels of Mg2+ switch neuronal firing from ictal-like bursting to status epilepticus [30]
Note. Adapted from “Transient incubation of cultured hippocampal neurons in the absence of magnesium induces rhythmic and synchronized epileptiform-like activity,” by Mele M, Vieira R, Correia B, De Luca P, Duarte FV, Pinheiro PS, et al. Sci Rep. 2021;11:11374 (https://www.nature.com/articles/s41598-021-90486-y). CC BY.
Epileptiform oscillations typically exhibit Ca2+-dependent synchronization throughout the network connections [41], which is reflected in the in-phase action potential generation and activity correlation, which is determined by the number of active synapses and is used to estimate the degradation of neuronal connections [42]. Analysis of optically imaged Ca2+ signals from synchronous oscillations has been proposed to characterize antiseizure medications in rodent culture [43].
Disadvantages of primary cortical cultures include variability in the spontaneous activity of the culture depending on the density of neuronal seeding [44]. To maximize spontaneous interictal activity in antiseizure medication testing, cultures with a density of 1,000–1,600 cells/mm2 are suggested [45, 46].
Organotypic cultured rodent brain slices
The ex vivo culture of neural tissue allows researchers to perform long-term experiments on neurons in situ with some degree of convenience, similar to the culture of primary dissociated neurons. In addition, the structure and arrangement of the cells are similar to that of the original brain area that served as the donor [36]. Slices show spontaneous activity after a certain incubation time [47]. In organotypic slice cultures, neurons form altered connections between each other within the overall neural network in response to deafferentation and significant disruption of local connections and projections induced by the slicing procedure. These phenomena are believed to be similar to those underlying epileptogenesis in post-traumatic epilepsy [10]. After 14–21 days of in vitro culture, a significant proportion of organotypic slices of the CA3 region of the rat hippocampus demonstrate spontaneous epileptiform discharges that are classified as interictal and ictal-like [11]. The proportion of cultures showing ictal events increases steadily with incubation time. In terms of sensitivity to anticonvulsants and a steady increase in excitability, such in vitro activity resembles in vivo epilepsy, although it shows a higher increase in the frequency of seizure events over time [10].
In recent epilepsy studies, organotypic cultures have been used to record intracellular chlorine and pH dynamics using genetically encoded sensors during seizure activity [48]. Genetic targeting methods allow the separation of chlorine and pH change signal recordings between specific groups of neurons, such as pyramidal neurons and parvalbumin/somatostatin-positive interneurons [48]. Under incubation conditions, multiple spontaneous activities and synchronous bursts resembling interictal spikes can be recorded after 14 days in vitro, while synaptogenesis de novo is taking place [11]. Interictal discharges precede the appearance of epileptiform activity on the 21st day in vitro when 50% of organotypic hippocampal slice cultures exhibit sustained epileptiform discharges. Thus, organotypic hippocampal slice cultures represent a useful in vitro model system to study the mechanisms of epileptogenesis for the screening of antiseizure medications and seizure-provoking drugs in reduced preparations.
Organotypic cultured human brain slices
The development of post-mortem human brain culture protocols has been reported that allow long-term viability of living human brain tissue in vitro for up to 30 days or more with strong degradation of pyramidal neurons [49]. It is possible that pyramidal cell degradation is caused by damage to their dendritic tree during extraction of small brain fragments. In addition, brain slices are often obtained from surgically treated patients with pharmacoresistant epilepsy, so they have a history of pharmacological exposure during treatment trials [50], so their availability and usability are quite limited. Hippocampal or neocortical tissue is most commonly cultured [51]. On the other hand, the presence of microglia and other glial cells allows life-stage sensitive assessment of brain responses, including aging [52, 53]. It has also been possible to culture adult post-mortem brain tissue [49] and embryonic aborted material [54]. When cultured, hippocampal and neocortical slices develop spontaneous electrical events similar to interictal discharges [55], and it is also possible to pharmacologically induce convulsive ictal seizure events with convulsants [50], a model in which experimenters test potential antiseizure medications. However, acute human brain slices are most commonly used in epilepsy-related studies, such as models of spreading depression [56].
Human secondary stem-cell-derived cultures
Recently, approaches that allow the generation of neurons from human somatic cells (usually fibroblasts) via induced pluripotent stem cells (iPSCs) using organoid culture have become widespread [57]. Potential advantages of such approaches include the possibility to experiment with neurons from patients with epileptic disorders caused by known epileptogenic mutations without invasive techniques or damage to the patient’s brain, allowing a more personalized treatment in the future. In addition, direct neuronal reprogramming methods have recently been proposed to convert glia into neurons without going through a pluripotent state [58]. However, despite the vast amount of methodological work, its current implementation has obvious drawbacks that practically offset its advantages. These include the inability to derive functional neurons with a sufficient degree of differentiation and/or maturity, resulting in an order of magnitude higher input impedance and compromised action potential amplitudes [24, 59–61]. Perhaps the biggest obstacle here is the large time scale on which human neurons develop, which typically takes years, as opposed to the developmental scale of rodent neurons, which is measured in weeks [61]. In addition, although initially neuronal culture derived from iPSCs demonstrated mixed glutamate- and GABAergic neurons, upon maturation culture can degrade to glutamatergic-only neurons [60]. Importantly, co-culture experiments with iPSCs-derived cell types showed that synchronized epileptiform oscillations developed only when both glutamatergic and GABAergic neurons were co-cultured with astrocytes [57].
To date, the best results in terms of action potential characteristics, input resistance (negatively influenced by the number of channels), and synapse-dependent synchronization have been obtained using the most complex and time-consuming protocols based on 3D-embryoid body culture and directed cell differentiation for up to 6 months [61]. Attempts have also been made to overcome the developmental delay by xenotransplanting differentiated human neurons into young rat brains [62], but it remains unclear how feasible it is to use neurons differentiated in this way as part of chimeric rodent brain tissue for epilepsy research.
Despite serious obstacles, pioneering works in differentiated human neurons are emerging. A multi-electrode array integrated into a culture dish has been reported as a model of pharmacologically-induced seizures for antiseizure medication screening using electrophysiological recording and functional imaging of neuronal activity [18, 63].
Stem-cell therapy for epilepsy
Stem cell technologies have also been proposed to alleviate epilepsy symptoms in therapeutic approaches involving the injection of derivatives of these cells into the body or directly into the brain. A number of model studies have been reported in recent years, most using programmed differentiation into additional inhibitory interneurons that have integrated into the central nervous system and have reduced the severity and consequences of epilepsy in rodent models [64, 65]. Besides interneurons, differentiation into paracrine cells serving as a source of neurotrophic factors was used [66, 67]. Stem cells of mesenchymal origin have already been used in experimental treatments of individual epilepsy patients, with at least initial improvements [68, 69]. However, it is not precisely defined into which cell type they differentiate [65]. When injected into rodent brains, human-iPSCs that mature into GABAergic inhibitory neurons reduce symptom severity in an experimental epilepsy model [70, 71]. A detailed review of the perspectives of stem cell therapy in the treatment of epilepsy is provided by Alayli et al. [64].
Conclusions
Cell cultures in vitro produce spontaneous epileptiform discharges while prolonged synchronized seizures and status epilepticus can also be induced. Therefore, a reduced model of brain tissue is feasible either for studying basic cellular mechanisms of epilepsy or for testing new antiseizure medications or genetic therapeutic vectors in a well-controlled environment using stationary methods for recording and imaging cellular activity in monolayers or oligolayers. Not only do culture methods provide relatively simple and inexpensive rodent models, but they also provide quite sophisticated human brain slice cultures and, more importantly, stem cell differentiated neurons, which have great potential for personalized in vitro epilepsy studies and stem cell-based therapy of epileptic disorders. Thus, a variety of cell culture models are available to address needs ranging from basic experiments to study network excitability mechanisms to non-invasive genetic experiments to treat human patients.
Abbreviations
iPSCs: |
induced pluripotent stem cells |
Declarations
Author contributions
IO: Writing—original draft, Formal analysis. OI: Methodology. PB: Conceptualization, Supervision, Funding acquisition. EN: Conceptualization, Methodology, Writing—original draft, Writing—review & editing.
Conflicts of interest
The authors declare that they have no conflicts of interest.
Ethical approval
Not applicable.
Consent to participate
Not applicable.
Consent to publication
Not applicable.
Availability of data and materials
Not applicable.
Funding
This research was funded by the Russian Science Foundation; grant [RSF 20-15-00408]. The funders had no role in study design, data collection and analysis, decision to publish, or preparation of the manuscript.
Copyright
© The Author(s) 2024.