Abstract
The prevalence of metabolic dysfunction-associated fatty liver disease (MAFLD) is increasing rapidly worldwide due to the obesity epidemic. Advanced stages of the MAFLD, such as non-alcoholic steatohepatitis (NASH) with advanced fibrosis or cirrhosis are affecting global health. Extracellular vesicles (EVs) are released by all cell types and are important in cell-to-cell communication and maintaining homeostasis, but they also play a role in the pathogenesis of various diseases. EVs contain biological information such as lipids, proteins, messenger RNAs (mRNAs), small RNAs, and DNA, and they act on (distant) target cells. The cargo of EVs is dependent on the type and the state of the releasing cell. EVs have been proposed as biomarkers, prognostic, and even therapeutic agents, also in the context of liver diseases. This review aims to give an overview of the current knowledge on EVs in MAFLD, including the role and interaction of EVs with different cell types in the liver. Several aspects of EVs, including their origin, characteristics, cargo, and functions are reviewed. Moreover, the potential of EVs as targets for the treatment of MAFLD is discussed.
Keywords
Extracellular vesicles, exosomes, metabolic dysfunction-associated fatty liver disease, non-alcoholic steatohepatitis, lipotoxicity, liver fibrosis, biomarkers, therapeutic applicationIntroduction
Metabolic dysfunction-associated fatty liver disease (MAFLD) is considered one of the most important causes of chronic liver disease worldwide. The prevalence in Western countries ranges from 20% to 30% in the general population. It is estimated that in the near future the number of cases will continue to rise because of the worldwide increased prevalence of obesity [1]. MAFLD is the result of impaired lipid metabolism resulting in an excessive accumulation of lipids in hepatocytes. Furthermore, it is associated with manifestations of metabolic syndrome such as type 2 diabetes, atherosclerosis, dyslipidemia, and hypertension [2]. About 3% to 5% of patients with MAFLD have non-alcoholic steatohepatitis (NASH), which is characterized by chronic inflammation, ballooning of hepatocytes, and progression to fibrosis [3]. NASH is also an important risk factor for the development of cirrhosis and even hepatocellular carcinoma (HCC). Drug-based therapy for MAFLD and its advanced stages is lacking. Currently, the only effective treatment for advanced MAFLD (cirrhosis) is liver transplantation or bariatric surgery [4]. Non-invasive, accurate tools to determine the stage of MALFD are lacking but urgently needed. Major efforts have been made to identify novel non-invasive biomarkers to diagnose and stage the MAFLD. In the past decades, research on extracellular vesicles (EVs) has evolved from basic research to clinical application. EVs were first described in 1946 by Chargaff and West [5] as pellets resulting from ultracentrifugation of blood plasma. EVs may have significant biological and clinical value as they reflect the stage of the cell releasing the EVs. EVs are nanometer to micrometer-sized particles and can be subdivided into three groups: exosomes, microvesicles, and apoptotic bodies (see section 2 for more details). The role of EVs in intercellular transport and communication with the discovery that EVs contain RNA was reported in 2006 [6]. The cargo of EVs is composed of messenger RNAs (mRNAs), microRNAs (miRNAs), DNA, proteins, and lipids that may contribute to (patho)physiological effects in target cells [7]. Proteins identified in EVs include pro-inflammatory cytokines, regulatory proteins, active peptides, and growth factors [8, 9]. The identification of biologically active components in EVs has sparked interest not only in their (patho)physiological role but also in their application in diagnosis and even therapy, including MAFLD, chronic hepatitis B virus (HBV) and C (HCV), HCC, and acute liver failure [10–13]. EVs released from injured hepatocytes can have effects on nearby healthy hepatocytes, non-parenchymal liver cells, e.g., Kupffer cells (KCs) and hepatic stellate cells (HSCs), as well as more distant non-hepatic cells [13–15]. These findings are particularly relevant for MAFLD, where growing evidence suggests that metabolic reprogramming and oxidative stress are key mechanisms that affect liver function in these conditions. This review aims to discuss the role of EVs in the context of MAFLD, with special emphasis on their role in intercellular communication and their potential application for diagnosis and treatment.
Classification and biogenesis of EVs
EVs are spherical particles enclosed by a lipid membrane [16]. They are generated by all cell types and can be detected in diverse biological fluids such as blood, saliva, urine, semen, breast milk, and others [17]. They are important mediators in cell-cell communication due to their biological characteristics: they are composed of membranes and cytosolic content also known as ‘molecular cargo’, containing bioactive molecules that can serve as messengers. The molecular cargo varies according to the cell of origin and the condition of the releasing cell. These bioactive molecules contain proteins [e.g., tetraspanins, cytoskeletal proteins, cytosolic proteins, cytokines, extracellular matrix (ECM) proteins, and plasma membrane proteins], lipids, and nucleic acids [e.g., miRNA, mRNA, ribosomal RNA, and cell-free DNA, including double-stranded (ds), and single-stranded (ss) fragments, mitochondrial DNA (mtDNA), extrachromosomal circular DNA and viral, bacterial and plasmid DNA] [13, 18–21], which can be transferred to target cells by membrane fusion. The molecular cargo of EVs can then cause (patho)physiological changes in target cells. Target cells can be nearby cells but also distant cells, generating responses at distant sites without the need for direct contact between cells [8].
EVs can be classified according to their size and biogenesis (Table 1). Three distinct groups are recognized: large EVs also known as microvesicles, ranging in size from 100 nm to 1 μm (diameter); small EVs or exosomes, with a diameter from 30 nm to 100 nm and apoptotic bodies ranging in size from 500 nm to 5,000 nm. Microvesicles are produced by the protrusion or outward budding of the plasma membrane into the extracellular space by multiple mechanisms involving evagination of the plasma membrane via the endosomal sorting complex required for transport-I (ESCRT-I) subcomplex [22], Rho family-induced cytoskeletal rearrangements [23], redistribution of phospholipids via intracellular calcium signaling [24], membrane curvature proteins (e.g., ESCRT-III proteins) [25] and phosphatidylserine aggregation [26]. Exosomes are derived from endosomal multivesicular bodies (MVBs) that, upon fusion with the plasma membrane, release intraluminal vesicles into the extracellular environment regulated in part by Rab subfamily of small GTPases (Rab protein) [27, 28]. This process is mediated by two mechanisms: the ESCRT-dependent vesicular pathway and an ESCRT-independent pathway [28, 29]. The first pathway is triggered by protein ubiquitination with ESCRT proteins guiding cargo selection, clustering, and membrane fusion to form intraluminal vesicles. The second pathway is driven by a process involving ceramides and tetraspanins [28, 29]. The last group of vesicles is the apoptotic bodies that are membrane-surrounded fragments of cells undergoing apoptosis [17], which are released from dying cells into the extracellular space [30].
Three major types of EVs and their characterization
EVs classification | Size | Biogenesis | Markers | Physical methods |
---|---|---|---|---|
Microvesicles | 100–1,000 nm | Plasma membrane budding | Integrins, selectins, and CD40 | SEM, TEM, AFM, NTA, TRPS, flow cytometry |
Exosomes | 30–100 nm | Endosomal MVBs | ESCRT proteins, ALIX, TSG101, HSP90, tetraspanins (CD81, CD63, CD9), Rab proteins, flotillin | SEM, TEM, Cryo-EM, AFM, DLS, NTA, TRPS, SEA, flow cytometry |
Apoptotic bodies | 500–5,000 nm | Membrane-surrounded cell fragments | Annexin V, thrombospondin, C3b | SEM, TEM, flow cytometry |
SEM: scanning electron microscopy; TEM: transmission electron microscopy; AFM: atomic force microscopy; NTA: nanoparticle tracking analysis; TRPS: tunable resistive pulse sensing; Cryo-EM: cyro-electron microscopy; DLS: dynamic light scattering; SEA: single EV analysis; C3b: activated fragment of complement component C3: ALIX: apoptosis-linked gene 2-interacting protein X; TSG101: tumor susceptibility gene 101; HSP90: heat shock protein 90
To characterize and classify the EVs, specific markers have been identified, e.g., tetraspanins in exosomes and integrins in microvesicles. In addition, multiple analytical methods, notably electron microscopy and size determination, have been used to identify different types of EVs (see Table 1) [31].
EVs mediate cell-to-cell communication in MAFLD
In recent years, increasing evidence has been collected on the role of EVs in MAFLD and many studies support the hypothesis that EVs are involved in inflammation and fibrosis in MAFLD [7]. The excessive lipid accumulation can cause lipotoxicity in hepatocytes, triggering pro-inflammatory signaling and cell death [32]. Furthermore, in response to stress hepatocytes release large numbers of EVs, leading to the activation of non-parenchymal inflammatory cells in the liver, further promoting inflammation and fibrosis [29]. This is supported by the fact that in patients with NASH increased levels of circulating EVs have been observed [33].
The liver contains multiple cell types, including parenchymal cells (hepatocytes) and non-parenchymal cells (NPCs) such as HSCs, liver-specific macrophages, or KCs and liver sinusoidal endothelial cells (LSECs). Effective communication between different liver cell types ensures normal liver function and maintains hepatic homeostasis [34]. Likewise, impaired intercellular communication is involved in the pathogenesis of various liver diseases, including MAFLD [35]. EVs have been demonstrated to play an important role in intercellular crosstalk in the liver also in the context of MAFLD [34–36]. In this section, we will discuss the role of EVs in intercellular communication in the context of MAFLD (Figure 1 and Table 2).
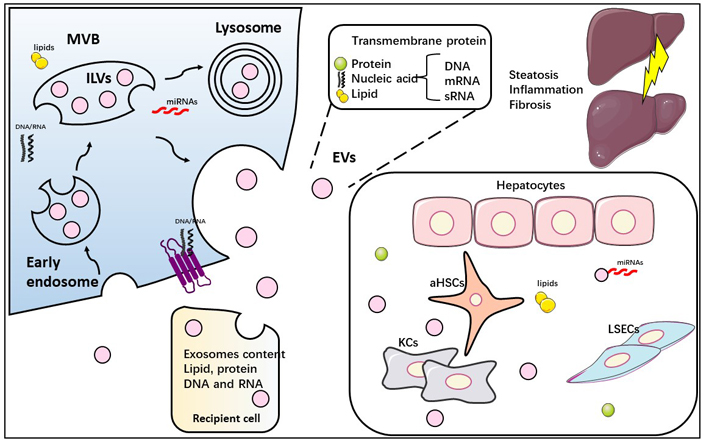
Schematic depiction of biosynthesis and release of exosomes and their role in cell-to-cell communication in the liver. Early endosomes are formed from the plasma membrane and subsequently transformed into MVBs (MVBs are formed by the invagination of endosomal membranes). They then fuse with lysosomes or with the plasma membrane. The resulting vesicles are degraded by lysosomes or secreted as exosomes. The cargo of exosomes is dependent on the cell type and the state of the cell. In liver injury, stressed hepatocytes release EVs containing DNA, RNA, proteins, and lipids that have a potent pro-inflammatory and pro-fibrogenic effect, activating both KCs as well as aHSCs. ILVs: intraluminal vesicles; aHSCs: activated HSCs; sRNA: small RNA
Overview of studies demonstrating EV-mediated cell-to-cell communication in the liver in MAFLD
Cell source | Cargo | Target cell | Associated molecules and/or signaling pathways | Role in MAFLD | References |
---|---|---|---|---|---|
Hepatocytes | Not evaluated | KCs | Not evaluated | Pro-inflammatory | [37] |
TRAIL | Bone marrow-derived macrophages (BMDM) and THP-1 macrophages | CHOP-DR5-Caspase-3/Caspase-8-ROCK1NF-κBRIP1 | Promotes M1 polarization of BMDM and THP-1 macrophages | [14] | |
ITGβ1 | THP-1 macrophages | Not evaluated | Promotes THP-1 inflammatory phenotype and increasesTHP-1-LSEC adhesion | [38] | |
Ceramides | Macrophages | IRE1α-XBP1-Sptlc1 and Sptlc2 | Promotes activation and recruitment of macrophages | [11, 39] | |
S1P | Macrophages | SphK1 and SphK2 S1P1 receptor | Promotes activation and recruitment of macrophages | [40] | |
mtDNA | KCs and RAW 264.7 cells | TLR-9 | Promotes activation of KCs and RAW 264.7 cells | [41, 42] | |
HSCs | Proteins | Not evaluated | ECM components, proteasome, collagens, vesicular transport, metabolic enzymes, ribosomes, and chaperones | Regulation of HSC activation and fibrogenic pathways in vivo | [43] |
Fibrogenic proteins | HSCs, macrophages, and endothelial cells (ECs) | PDGF; SHP2; mTOR signaling; ROCK signaling | Autophagy plays a critical role in the release of pro-fibrogenic EVs and HSC function | [44] | |
LSECs | IGFBP7; ADAMTS1 | Fibrotic Th17 cells | Histone deacetylase 2 (HDAC2)DNA methyltransferase 1 (DNMT1) | Epigenetic dysregulation of LSEC exacerbates liver disease | [45] |
Tropomyosin-1 (TPM1) | Rat hepatocytes, HSCs, human macrophages | ESCRT-dependent pathway (Mvb12-a, Mvb12-b), lipid-rafts structures such as Flot2, tetraspanins CD9 and CD81, and the Rab3 small GTPase | Deactivation of HSCs | [46] |
TRAIL: tumor necrosis factor-related apoptosis-inducing ligand; ITGβ1: integrin β1; IGFBP7: insulin-like growth factor-binding protein-7; Th17: T helper type 17; ROCK1: Rho-associated, coiled-coil-containing protein kinase 1; NF-κB: nuclear factor-κB; RIP1: receptor-interacting protein kinase 1; IRE1α: inositol-requiring transmembrane kinase/endoribonuclease 1α; SphK1: sphingosine kinase 1; TLR-9: Toll-like receptor-9; PDGF: platelet-derived growth factor; SHP2: srchomology-2-domain-containing PTP 2; mTOR: mechanistic target of rapamycin; CHOP: C/EBP homologous protein; DR5: death receptor 5; ADAMTS1: ADAM metallopeptidase with thrombospondin type 1 motif 1; XBP1: X-box binding protein 1; Sptlc1: serine palmitoyltransferase long chain base subunit 1; S1P: sphingosine 1-phosphate; Flot2: flotillin 2; Mvb12-a/b: multivesicular body subunit 12A/B
Hepatocyte-derived EVs in MAFLD
Hepatocytes are the most abundant cells within the liver [34] and play an important role in liver-specific functions like glycogen metabolism, bile secretion, lipid metabolism, steroid hormone metabolism, plasma protein synthesis, and detoxification.
Many studies have shown that EVs released from lipotoxic hepatocytes promote inflammation in MAFLD [11, 14, 37–42, 47–49]. Moreover, hypoxic conditions, present in MAFLD, aggravate the pro-inflammatory effect of EVs derived from lipotoxic hepatocytes on KCs [37]. Palmitate and lysophosphatidyl choline (LPC) treatment increased the release of EVs enriched with TRAIL from hepatocytes. This release was mediated by the CHOP-DR5-Caspase-8/Caspase-3-ROCK1 signaling cascade. TRAIL-enriched EVs induced the activation of various types of macrophages. On the one hand, they change the polarization of mouse BMDM towards a classical M1 macrophage phenotype with increased expression of interleukin-1β (IL-1β) and IL-6 in a DR5-dependent manner. On the other hand, human THP-1 macrophages treated with TRAIL-containing EVs were activated in an NF-κB and RIP1-dependent manner, and activation was inhibited by RIP1 inhibitor or loss of RIP1. Moreover, the application of a ROCK1 inhibitor in murine NASH models reduced the release of proinflammatory EVs and thus ameliorated NASH [14]. LPC-treated hepatocytes release active ITGβ1-enriched EVs that were shown to induce macrophage chemotaxis. ITGβ1-containing EVs mediate THP-1 cell adhesion to LSECs [38]. In addition, EVs enriched with ITGβ1 promote the inflammatory phenotype of monocytes, underscoring their proinflammatory role in MAFLD [38]. Several reports highlighted the role of lipotoxic ceramides and S1P in macrophage chemotaxis and inflammation in MAFLD [11, 39, 40]. EVs from lipotoxic hepatocytes enriched in ceramides induce the recruitment of macrophages into the liver and promote inflammation. The release of EVs enriched in ceramides is mediated by IRE1α and decreasing levels of IRE1α in hepatocytes decrease their release of EVs [11]. EVs released from palmitic acid-treated hepatocytes also promote the recruitment of macrophages into the liver via the interaction of exosomal S1P with the S1P receptor in macrophages. Inactivation of S1P signaling by preventing sphingosine phosphorylation via inhibition of SphK1 and SphK2 or deletion of the S1P receptor in macrophages results in the loss of the chemoattractive effects of EVs derived from palmitate-treated hepatocytes on macrophages [40].
Finally, EVs from hydrogen peroxide-damaged hepatocytes are enriched in mtDNA that increase the expression of pro-inflammatory cytokines on murine RAW 264.7 cells in a TLR-9 dependent manner, whereas IL-22Fc pretreatment of these hepatocytes decrease the pro-inflammatory potential of these EVs [42].
EVs released from non-parenchymal liver cells in MAFLD
Hepatic fibrosis is characterized by the excessive deposition of ECM in the liver. The main producers of ECM in the liver are aHSCs. The HSCs reside in the space of Disse. Under normal conditions, HSCs are quiescent pericyte-like cells. In chronic liver injury, HSCs transdifferentiate into an activated phenotype, characterized by loss of lipid stores, increased synthesis and secretion of ECM components, and increased proliferation. Activation is also associated with a 4.5-fold increase in EV production [43, 44]. Recent studies have shown that exosomes containing specific miRNAs from lipotoxic hepatocytes can promote stellate cell activation, proliferation, and migration [13, 15]. A comparative proteomic analysis of mouse HSC-derived EVs indicated that EVs from HSCs may play a key role in the regulation of HSC function and activation in vivo [43]. The internalization of hepatocyte-derived EVs by HSCs is dependent on the presence of either vanin-1 (VNN-1) or fibronectin (FN) [43, 50, 51]. Once internalized, these EVs stimulate the expression of pro-fibrotic mediators in HSCs, as also observed in our studies: hypoxic, palmitate-treated HepG2 cells release EVs that increase the expression of transforming growth factor-β (TGF-β), connective tissue growth factor (CCN2), collagen type 1 and α-smooth muscle actin (α-SMA) in HSCs [43, 52]. In contrast, EVs secreted by quiescent HSCs contain high levels of Twist 1, which drives miR-214 expression and results in the suppression of fibrogenic signaling. It is therefore relevant to consider the activation state when investigating HSC-derived EVs [43, 53, 54]. EVs can also act in an autocrine manner on HSCs: CCN2 promotes activation of HSCs and was detected in EVs released by HSCs [55].
LSECs are a major component of liver NPCs [56, 57]. Whereas vascular ECs form a continuous lining in arteries and veins, LSECs form a discontinuous layer in liver sinusoids. Moreover, in contrast to vascular ECs, LSECs are fenestrated [45, 46]. In chronic liver diseases like NASH, the LSECs dedifferentiate and form a continuous lining and lose their fenestrae. One study [45], using single-cell omics analysis, demonstrated that epigenetic dysregulation of LSECs promotes the dedifferentiation of these cells from a sinusoidal to a vascular phenotype. This was associated with increased production of EVs containing IGFBP7 and ADAMTS1, which further promoted the recruitment of pro-fibrogenic Th17 cells to the liver and exacerbation of hepatic fibrosis [45]. Investigation of the proteome of LSEC-derived EVs during the progression of chronic liver disease demonstrated that LSEC-derived EVs contain high levels of angiocrine effectors and tropomyosin 1. Subsequently, tropomyosin was shown to have a de-activating effect on HSCs [46].
KCs are the liver-specific macrophages. They play an important role as inflammatory cells in the progression of chronic liver diseases and secrete immunosuppressive factors and metabolites, however, not much is known about the role of KC-derived EVs in MAFLD [58, 59].
Signaling pathways regulated by EVs in the context of MAFLD
Recent evidence suggests that EVs are important regulators of the inflammatory response (Table 2) and it has been proposed that proinflammatory EVs contribute to MAFLD progression [33]. The role of EVs in the pathophysiological mechanisms during MAFLD progression is discussed in this section.
Hepatic steatosis and lipotoxicity
Steatosis is the accumulation of lipids in hepatocytes and is the earliest and defining feature of MAFLD. Studies in mice have shown that EVs are involved in the response to lipid overload in the liver and that the liver can control lipid remodeling in adipose tissue via EVs in conditions of steatosis [60]. Recent findings suggest that EVs can be mediators of lipotoxicity during MAFLD [32]. Lipid accumulation and in particular lipotoxicity can trigger cell signaling pathways that favor the release of EVs from hepatocytes [7]. In vitro studies using primary mouse hepatocytes and human Huh7 cells have shown that palmitate activates DR5 signaling, which induces the release of EVs that promote the inflammatory phenotype of macrophages [14]. Lipotoxicity induced by saturated fatty acids like palmitate increases membrane lipid saturation causing endoplasmic reticulum (ER) stress. Lipotoxicity has also been shown to induce membrane alterations in the EV membrane and EV lipid cargo, suggesting that these EVs might be useful to track lipotoxic stress in the liver [61].
NASH, the inflammatory stage of MAFLD, is characterized by increased levels of pro-inflammatory and chemotactic cytokines, oxidative stress, mitochondrial and ER stress, lipid peroxidation, and eventually cell death. In particular oxidative stress is considered a central mechanism in the pathophysiology of MAFLD [62]. Lipotoxicity can induce oxidative stress and inflammatory response by increasing lipid peroxidation and the formation of neo-epitopes such as oxidation-specific epitopes (OSEs) [63]. These OSEs have been demonstrated to be present in EVs as well as in circulating lipoproteins and can contribute to the activation of other liver cells such as KCs, promoting inflammation [64]. Lipotoxic stress is also related to organelle dysfunction, including ER stress and mitochondrial dysfunction. In a murine model of diet-induced NASH, mitochondrial stress and oxidative stress correlate with increased release of EVs, containing oxidatively damaged mitochondrial particles. These EVs can contribute to an antioxidant response to protect from acute oxidative stress but they can also be deleterious by enhancing the inflammatory response [65].
Inflammatory response
The innate immune response plays an important role in inflammation. Classical innate immune receptors include pattern recognition receptors (PRRs) like the TLRs, which detect damage-associated molecular patterns (DAMPs). Activation of TLRs has been demonstrated in MAFLD [66]. mtDNA in EVs released from damaged hepatocytes activates TLR-9 on target cells [41, 42]. Loss of TLR-9 attenuates NASH and specific deletion of TLR-9 in KCs reduced the expression of inflammatory cytokines such as IL-β, IL-6, and tumour necrosis factor α (TNFα) in a high-fat diet (HFD) model of MAFLD [41]. Hepatocytes from HFD hepatocytes release EVs enriched in mtDNA, resulting in TLR-9 activation and increased expression of the TLR-9 downstream target TNFα in KCs. Plasma from obese patients with liver injury contained higher levels of mtDNA and TLR-9 ligand activity, suggesting that mtDNA from damaged hepatocytes and TLR-9 activation is involved in the inflammatory response in NASH, possibly mediated via EVs [41]. EVs derived from hepatocytes mediate the activation of TLR-3 in HSCs. This may aggravate fibrosis by enhancing IL-17 production by γδ T cells at the early stages of liver fibrosis [67].
Other pro-inflammatory PRRs associated with the development of MAFLD include the inflammasome sensor molecule typically a NOD-like receptor (NLR) family pyrin domain containing protein 3 (NLRP3), which is a component of inflammasomes and has been shown to promote inflammation and fibrogenesis during the progression of MAFLD [68]. EVs modulate the activation of NLRP3 [69]. In the choline deficient L-amino acid (CDAA) and HFD mice models of MAFLD, altered levels of proteins related to cell death, stress, and angiogenesis were demonstrated in plasma-derived EVs [69]. Other studies showed the enrichment of miR-7 in hepatic EVs that promote lysosomal membrane barrier permeability, inducing the activation of NLRP3 and microvascular hyperpermeability [70]. Furthermore, it has been shown that steatotic hepatic cells release EVs that induce the expression of NLRP3 and inflammasome activity in target cells, including macrophages. Moreover, in this study increased hepatic expression of NLRP3 was detected in mice with methionine-choline deficient diet-induced NASH [71].
In addition to macrophages, ECs are also a target for EVs. It has been demonstrated that human umbilical vein ECs (HUVECs) can internalize EVs derived from palmitic acid-treated human HepG2 cells and this process appeared to be dependent on the presence of lipid rafts and VNN-1. In fact, VNN-1 is not only involved in the internalization of EVs, but also in EC migration [72]. Another study demonstrated uptake of EVs derived from palmitic acid-treated human Huh7 cells by HUVEC cells, leading to increased expression of inflammation-related genes in HUVECs like E-selectin, intercellular adhesion molecule-1 (ICAM-1), vascular cell adhesion molecule-1 (VCAM-1), IL-1β and matrix metalloproteinase-1 (MMP-1) [73].
To achieve novel interventional and non-interventional strategies to treat patients with MAFLD, a thorough understanding of the mechanisms leading to MAFLD is necessary. It is clear from the available evidence that both paracrine and autocrine communication mediated by EVs play an important role in the pathogenesis of MAFLD. Therefore, more studies, focusing on analyzing the content and effects of the cargo of EVs are necessary.
Diagnostic and therapeutic application
EVs in MAFLD: interventional implications
Research on EVs has given us insights into the possible application of EVs as diagnostic tools. In 2011, the presence of small EVs in plasma from patients with active hepatitis C was reported [74]. Interestingly, these microparticles were derived from CD4+ and CD8+ T cells. Moreover, these EVs induced the expression of several metalloproteases such as MMP-1, MMP-3, MMP-9, and MMP-13 and reduced the expression of procollagen α1(I) mRNA in the human HSC cell line LX-2 [74].
In 2012, it was demonstrated that patients with NASH have increased plasma levels of circulating EVs derived from invariant natural killer T (iNKT) cells and monocytes (CD14+), which were also postulated to play a role in the pathogenesis of NASH [10]. Taken together, these results indicate that the amount and content of circulating EVs can provide information on the clinical status.
Since the pathophysiology of MAFLD involves hepatocyte lipotoxicity and concomitant pro-inflammatory and profibrogenic responses, the macrophage (KC) and the HSC are attractive targets for intervention. Adipose-derived stem cells (ADSCs) secrete EVs that promote M2 polarization in macrophages (high Arg-1 phenotype), with beneficial effects on inflammation, systemic insulin resistance, dyslipidemia, and hepatic steatosis in an in vivo model of NASH [75]. Stem cells (SCs) may be a suitable medium to deliver EVs to target cells. SCs are cell types that can self-renew and differentiate into other cell types. Furthermore, it has been proposed that EVs derived from SCs may induce regenerative programs in injured organs such as the liver by transcriptional or translational modification [76]. EVs from human liver SC (HLSC; HLSC-EVs) improved liver function in a murine diet-induced NASH model [77]. In addition, the transcriptomic analysis demonstrated 29 pro-fibrogenic genes (α-SMA, Col1α1, Ltbp-1, and TGF-β1) and pro-inflammatory genes [TNFα, IL-1β, interferon-γ (IFN-γ)] to be upregulated in hepatic tissue in NASH. Interestingly, the expression of 28 of these genes was significantly reduced after treatment with the HLSC-EVs [77]. Similarly, in a genetic model of NASH (Mc4r knockout mice), treatment with human adipose tissue-derived mesenchymal SC EVs (AT-MSC-EVs) significantly attenuated liver fibrosis. Moreover, different metabolic parameters such as triglyceride (TG), free fatty acids (FFAs), aspartate transaminase (AST), alanine transaminase (ALT), and albumin (ALB), glucose (Glu) or total bilirubin (T-bil) levels were also improved [78].
Since EVs have been shown to have therapeutic value in the resolution of MAFLD and since miRNAs are components of the cargo of EVs and miRNAs are involved in various biological functions, we will discuss the application of EV-derived miRNAs in more detail in the next section.
miRNAs loaded EVs as the potential therapeutic application in MAFLD
miRNAs have been implicated in the epigenetic regulation of cell proliferation, fat metabolism, and cell death [79]. Moreover, the role of miRNAs in liver inflammation, fibrosis, and cirrhosis has been acknowledged [80]. miRNAs are detected in biological fluids like blood, urine, tears, saliva, and breastmilk and can be used as diagnostic biomarkers, e.g., in type 2 diabetes, HCC, and MAFLD [81, 82]. miR-122 is released from damaged hepatocytes in alcoholic liver disease, viral hepatitis, and drug-induced liver injury [83–85]. miRNAs constitute an important class of gene-regulatory molecules in both humans and mice [86]. They are small, non-coding RNAs (18–25 nucleotides) and can be incorporated into exosomes and secreted into the extracellular environment [87]. miRNAs are involved in a number of biological functions such as cellular differentiation, proliferation, metabolism, and apoptosis [88, 89]. They regulate gene expression in a posttranscriptional manner via binding complementary mRNA transcripts, suppressing gene expression via mRNA degradation and repression of translation. miRNAs not only interact with mRNAs but also with long non-coding RNAs (lncRNAs), pseudogenes, and circular RNAs (circRNAs) [90]. Several miRNAs have been detected in liver-derived exosomes, e.g., miR-21, miR-125, miR-146, miR-192, miR-223, and miR-378 [91]. Exosomal miRNAs originating from lipotoxic hepatocytes are also crucial in inflammation during the progression of MAFLD [47, 48]. EVs from lipotoxic hepatocytes contain miR-192-5p. This miRNA was able to polarize THP-1 macrophages towards the pro-inflammatory M1 phenotype via targeting the 3’ untranslated region (3’ UTR) of Rictor and suppressing its expression, subsequently inhibiting downstream protein kinase B/forkhead box O1 (Akt/FoxO1) factors. Overexpression of miR-192-5p and lack of Rictor in THP-1 cells further underscored the key role of miR-192-5p in M1 macrophage polarization [47]. Oxidized low-density lipoprotein (ox-LDL) and cholesterol impaired degradation of MVBs in Huh7 cells, leading to increased release of EVs containing miR-122-5p, promoting M1 polarization of THP-1 macrophages [49]. The M1 polarization proved to be miR-122-5p dependent since downregulation of miR-122-5p in Huh7 cells abrogated the exosome-induced M1 polarization of THP-1 macrophages [49]. miR-128-3p was identified as the exosomal messenger that reduced the expression of the stellate cell quiescence marker peroxisome proliferator-activated receptor-γ (PPAR-γ) [15].
The expression of specific miRNAs was increased in EVs released from hepatocytes treated with toxic lipids, including miR-24, 19b, 34a, 122, and 192. High levels of exosomal miR-192 promote HSC proliferation and activation [13]. Increased circulating exosomal miR-192 and miR-122 in MAFLD patients further demonstrate that the miRNA profile may be a representative marker in the diagnosis of fibrosis in MAFLD [13].
Several studies indicated the therapeutic potential of exosomal miRNAs. Thus, miR-627-5p overexpressed in EVs from human umbilical cord-derived mesenchymal SCs (HUC-MSC-EVs) effectively reduced serum levels of ALT, AST, TG, and total cholesterol as well as lipid deposition in a high fat-high fructose diet rat model of NASH [92]. Moreover, in in vitro studies, miR-627-5p HUC-MSC-EVs improved cell viability and lipid homeostasis and inhibited apoptosis and fatty acid synthesis in palmitate-treated L-O2 cells [92]. Another relevant miRNA is miRNA-96-5p. Its presence in EVs from bone marrow-derived mesenchymal SCs (BM-MSC-EVs) downregulated fatty acid synthesis and lipid uptake in an HFD rat model of NASH, whereas mitophagy genes [Parkin, phosphatase and tensin homologue (PTEN)-induced putative kinase 1 (PINK1), unc-51 like autophagy activating kinase 1 (ULK1), B-cell lymphoma 2/adenovirus E1B 19 kDa protein-interacting protein 3 (BNIP3L)] and autophagic genes [autophagy related gene 5 (ATG5), ATG7, ATG12] were induced [93]. Liver-derived EVs also have an important role in lipid metabolism. Wu et al. [94] demonstrated, using a murine diet model of MAFLD, that miR-130a-3p regulates Glu metabolism, suppressing PH domain leucine-rich repeat protein phosphatase 2 (PHLPP2) expression and activating Akt-A160-Glu transporter type 4 (GLUT4) signaling in adipocytes, thus increasing Glu uptake. The role of the liver in regulating lipid metabolism, in particular, whether hepatic EVs containing miRNAs play a role in the communication between liver and adipose tissue, was also investigated in a murine MAFLD model [60]. In this study it was shown that Let-7e-5p enhances adipocyte lipid deposition by increasing lipogenesis and inhibiting lipid oxidation via PPAR-γ co-activator 1-alpha (PGC1α), confirming that the liver orchestrates an integrated response involving EVs to modulate lipid metabolism.
In conclusion, EVs as an interventional therapy still represent a largely unexplored field. Nevertheless, promising results in the treatment of MAFLD as well as other liver diseases have been reported. Due to the heterogeneity of EVs, diverse effects on target cells have been reported. This stresses the need to standardize the use of EVs in therapeutic applications (isolation, characterization, standardization of administration, etc.) and to take into account the type of the EV-releasing cell, the state of the releasing cell, the stimulus that led to their secretion, their cargo, as well as the target cell, in order to be able to carry out clinical trials in the near future (see Table 3).
Diagnostic and therapeutic potential of EVs in MAFLD
Cell source | Target cell | EVs markers | Biological effects | References |
---|---|---|---|---|
Hepatocytes | HSCs | TLR-3 | Pro-fibrotic response at early stages of liver fibrosis | [67] |
Liver (circulating EVs) | Not evaluated | miR-122, miR-192 | Increased levels of circulating EVs are associated with the severity of MAFLD | [69] |
Hepatocytes | ECs (HUVECs) | VNN-1 | FFA-treated hepatocytes released EVs with proangiogenic effects | [72] |
ECs | HSCs | FN, integrin | HSC adherence and migration | [51] |
HSCs | Not evaluated | Twist 1 | Limits the magnitude of the fibrotic response | [54] |
CD4+ and CD8+ T from hepatitis C patient | HSCs | Not evaluated | Induce the upregulation of fibrolytic genes in HSCs | [74] |
Activated and quiescent HSCs | Not evaluated | CCN2 | HSC activation | [55] |
Adipocytes | HepG2 cells | Adipokines | Modulate insulin resistance | [95] |
ADSCs | KCs | Not evaluated | Polarize KCs toward Arg-1 M2 phenotype | [75] |
AMSCs | KCs | Not evaluated | Decreased number of Kupffer cells and decreased expression of inflammatory genes in NASH rat model | [96] |
HLSC | NASH rats | Not evaluated | Improved liver function with downregulation of pro-inflammatory and profibrotic genes | [78] |
Human adipose tissue-derived mesenchymal SCs | NASH mice | Not evaluated | Decreased liver fibrosis | [77] |
Hepatocytes | MacrophagesTHP-1 cells | miR-192-5pmiR-122-5p | Pro-inflammatory | [47, 49] |
Hepatocytes | HSCs and LX2 cells | miR-128-3pmiR-192 | Pro-fibrotic | [13, 15] |
Human umbilical cord-derived mesenchymal SCs | Rat NASH model | miR-627-5p | Improved liver function and reduced lipid accumulation | [92] |
Hepatocytes | Adipocytes | miR-130a-3pLet-7e-5p | Increased Glu uptake, lipogenesis, and decreased lipid oxidation | [60] |
KCs | Huh7 cells | miRNA-29 | Anti-HCV | [97] |
AMSCs: amnion-derived mesenchymal SCs
Conclusions and future prospects
MAFLD is a major global public health problem. It ranges from simple steatosis to advanced steatohepatitis (NASH) and can progress to fibrosis, cirrhosis, and even HCC. Much progress has been made in recent years to elucidate the mechanisms underlying the pathogenesis of MAFLD. Nevertheless, there is still an urgent need to develop (non-invasive) diagnostic, prognostic and therapeutic tools. The role and application of EVs is an emerging area of research. Not only the pathophysiological role of EVs in diseases is currently being studied, but also their application in diagnosis and as a potential tool for drug delivery.
In our review, we provide an overview of existing literature on EVs and their use as a therapeutic tool in the context of MAFLD. We conclude that EVs are intimately involved in the pathogenesis of MAFLD. They play an important role in cell-to-cell communication, not only within the liver but also at more distant sites (e.g., adipose tissue). EVs are crucial in the transfer of biological information and/or activity (e.g., mRNAs, miRNAs, proteins, enzymes, etc.) from the cell releasing the EV to the cell internalizing the EV. Since the cargo is dependent on the type of releasing cell and the state of these cells (e.g., lipotoxic vs. normal hepatocytes), the cargo and effect of EVs on targets cell will vary according to the type and state of the releasing cell.
There are still gaps in our knowledge on the role of EVs in general and in the context of MAFLD in particular. The effect of EVs from extra-hepatic tissues on liver cells in the context of MAFLD is largely unexplored (e.g., EVs from adipose tissue or atherosclerotic ECs). The use of SC-derived EVs as treatment needs to be further investigated and needs confirmation in clinical trials. The application of EVs in clinical trials also needs further exploration on the preparation, characterization, and administration of EVs as well as the manipulation of the cargo of EVs, e.g., by overexpressing specific therapeutic components (miRNAs). In this regard, it is also important to develop methods to identify the cellular source of EVs found in circulating plasma. So far, identification of the cellular source, using cell-specific markers in EVs, is only possible to some extent. Based on the existing knowledge and the high potential of EVs, as summarized in this review, we expect that research on EVs and their therapeutic application will expand significantly in the near future.
Abbreviations
ADAMTS1: | ADAM metallopeptidase with thrombospondin type 1 motif 1 |
aHSCs: | activated hepatic stellate cells |
ATG5: | autophagy related gene 5 |
BMDM: | bone marrow-derived macrophages |
CCN2: | connective tissue growth factor |
CHOP: | C/EBP homologous protein |
DR5: | death receptor 5 |
ECM: | extracellular matrix |
ECs: | endothelial cells |
ER: | endoplasmic reticulum |
ESCRT-I: | endosomal sorting complex required for transport-I |
EVs: | extracellular vesicles |
Glu: | glucose |
HCC: | hepatocellular carcinoma |
HFD: | high-fat diet |
HLSC: | human liver stem cell |
HSCs: | hepatic stellate cells |
HUVECs: | human umbilical vein endothelial cells |
IGFBP7: | insulin-like growth factor-binding protein-7 |
IL-1β: | interleukin-1β |
IRE1α: | inositol-requiring transmembrane kinase/endoribonuclease 1α |
ITGβ1: | integrin β1 |
KCs: | Kupffer cells |
LSECs: | liver sinusoidal endothelial cells |
MAFLD: | metabolic dysfunction-associated fatty liver disease |
miRNAs: | microRNAs |
MMP-1: | matrix metalloproteinase-1 |
mRNAs: | messenger RNAs |
mtDNA: | mitochondrial DNA |
MVBs: | multivesicular bodies |
NASH: | non-alcoholic steatohepatitis |
NF-κB: | nuclear factor-κB |
NLRP3: | NOD-like receptor family pyrin domain containing protein 3 |
Rab protein: | Rab subfamily of small GTPases |
RIP1: | receptor-interacting protein kinase 1 |
ROCK1: | Rho-associated, coiled-coil-containing protein kinase 1 |
S1P: | sphingosine 1-phosphate |
SCs: | stem cells |
SphK1: | sphingosine kinase 1 |
Th17: | T helper type 17 |
TLR-9: | Toll-like receptor-9 |
TNFα: | tumor necrosis factor α |
VNN-1: | vanin-1 |
Declarations
Author contributions
ZW contributed to the abstract, sections 1, 2, 5.2, conclusions and Figure 1. MX contributed to the
Conflicts of interest
The authors declare that they have no known competing financial interests of personal relationships that could have appeared to influence the work reported in this research paper.
Ethical approval
Not applicable.
Consent to participate
Not applicable.
Consent to publication
Not applicable.
Availability of data and materials
Not applicable.
Funding
This project is financially supported by the China Scholarship Council (CSC) grant 202008320321 (MX), grant 202006250036 (JW) and grant 201806170085 (ZW), Conacyt grant 795389 (MMA), Colciencias International Scholarship Program grant 783-2017 (JAO), the Abel Tasman Talent Program of the University Medical Center Groningen (MCTA), Dutch Digestive Foundation grant WO 21-03 (SSS; HB; HM). The funders had no role in study design, data collection and analysis, decision to publish, or preparation of the manuscript.
Copyright
© The Author(s) 2022.